Overview
This comprehensive article unifies an integrated analysis of five globally significant river basins—the Mekong, Mississippi, Amazon, Tana, and Rhine—through the lens of the water–energy–food–health–climate nexus. Each case study offers a detailed exploration of historical trajectories, critical transitions, and the evolving interplay between natural systems and human interventions. Our contributors, drawing on interdisciplinary research and diverse regional perspectives, examine how path dependencies, infrastructural legacies, and governance challenges have shaped the socio-ecological landscapes of these vital waterways.
The unified framework presented herein is designed to guide policymakers, researchers, and practitioners in understanding both shared challenges and unique regional dynamics. By highlighting key inflection points—from shifts in land use and hydrological management to the emergence of new technological and policy responses—this article aims to foster a deeper understanding of sustainable development strategies in the context of rapid environmental change. We invite readers to engage with the ensuing comparative analysis as a resource for advancing resilient, integrated approaches to river basin management worldwide.
The Case of Mekong River Basin
Lincoln Cheng
Historical Context of the Mekong Basin
Early Development and Path Dependencies: The Mekong’s socio-ecological landscape has been shaped by distinct historical phases. Mid-20th-century development plans laid groundwork for basin-wide cooperation, notably through the Mekong Committee (est. 1957) which coordinated investigations of the Lower Mekong Basin. However, decades of conflict and political upheaval slowed basin-scale development until the 1990s. The 1995 Mekong Agreement reconstituted cooperation as the Mekong River Commission (MRC), aiming for “sustainable development, utilization, management and conservation” of Mekong water resources. This marked a turning point: war-torn “battlefields” gave way to marketplaces, enabling foreign investment and large infrastructure projects.
Hydropower Boom: Since the 1990s, the Mekong Basin transitioned from a largely free-flowing river system to one increasingly impounded by dams. Early studies by the UN and US (ECAFE and Bureau of Reclamation) had identified hydropower potential, but Cold War tensions and limited financing delayed implementation. By the late 20th century, this changed dramatically. China began building its cascade of dams on the Lancang (upper Mekong), and the Asian Development Bank’s Greater Mekong Subregion program (since 1992) promoted cross-border power trade and dam construction. As of 2019, the Mekong Basin hosted 89 medium-to-large dams in the lower basin (with dozens more planned) and 11 large dams on the Chinese mainstream (with more under way). This hydropower boom set path dependencies: today’s water flows, sediment transport, and ecological dynamics are indelibly shaped by dam operations and reservoir management decisions spanning decades.
Land Use and Agriculture Transitions: Historically, the Mekong’s seasonal flood pulse supported an equilibrium between fisheries, flood-recession agriculture, and rice cultivation. Over time, deliberate shifts occurred. The Green Revolution introduced high-yield rice varieties and expanded irrigation, especially in Vietnam’s Mekong Delta – known as the nation’s “rice bowl”. By the 2000s, Vietnam intensified rice production to three crops per year in many areas, supported by dike construction to control floods and expanded groundwater pumping for irrigation. These choices boosted food production but at the cost of altered sediment flows and land subsidence, creating new vulnerabilities (explored in later sections). Logging and land conversion also transformed regional landscapes. In the 1970s, forests covered ~75% of the Greater Mekong; over the last 30 years, 30% of that forest cover disappeared, driven by agriculture, plantations, and timber extraction. This deforestation has reduced ecological resilience, affecting watersheds and carbon storage.
- Key Inflection Points: Major historical inflection points include:
- (a) 1995 – the Mekong Agreement’s signing, ushering in an era of institutionalized cooperation;
- (b) Early 2000s – China’s Mekong dams (e.g., Manwan, Dachaoshan) coming online, tangibly affecting downstream flows;
- (c) 2010–2012 – Laos’s decision to pursue mainstream dams (notably Xayaburi in 2012), testing regional commitments and sparking transboundary tensions;
- (d) 2016 – a record drought and salinity intrusion crisis in the Mekong Delta, linked to both El Niño and upstream dam water management, signaling the onset of acute climate impacts; and
- (e) 2020 – Cambodia’s moratorium on new Mekong mainstream dams through 2030, reflecting a policy pivot toward alternative energy and a recognition of hydropower’s socio-environmental costs. These turning points illustrate how past decisions – from dam building to land use change – have created path dependencies that condition present risk profiles.
Key Risk Factors in a WEFH Nexus
Water Risks
Hydrological Variability and Climate Impacts: The Mekong’s natural flow regime is highly seasonal, with monsoonal floods and dry-season lows. Climate change is amplifying this variability. In the past 50 years, the region warmed by 0.5–1.5 °C, and extreme events (droughts, floods, storms) are now more frequent. Models project continued warming and intensified hydrological extremes – wetter rainy seasons (risking floods) and drier dry seasons (worsening water shortages). Moreover, the timing of monsoon onset is shifting, undermining farmers’ and fishers’ ability to plan. These changes heighten uncertainty for water management, irrigation scheduling, and flood control infrastructure.
Upstream Dams and Flow Regulation: Rapid dam construction has introduced artificial flow regulation as a dominant force. China’s 11 mainstream dams (with 11 more planned) and scores of tributary dams can store billions of cubic meters. During droughts, dam operators may withhold water to maintain reservoir levels for power generation. Conversely, in wet season, dams trap floodwaters, sediment, and fish that would naturally replenish downstream floodplains. The cumulative effect is a dampened flood pulse and prolonged low-flow periods, directly impacting the Tonle Sap’s flood reversal and the Mekong Delta’s sediment deposition. The Mekong River Commission estimated that by 2020 only one-third of the Mekong’s historical sediment load was reaching Vietnam’s delta, with potential collapse to <5 million tonnes/year by 2040 if current trends continue. Reduced sediment means riverbank erosion, sinking delta lands, and less nutrient renewal for agriculture. Collectively, water risks manifest as increased drought/flood damage, unpredictable water availability for farms and cities, and destabilized riverine ecosystems.
Water Scarcity and Quality: Population growth, agriculture, and urbanization drive rising water demand, straining resources in the dry season. Groundwater overuse in the Mekong Delta, for example, contributes to land subsidence at ~1 cm/year on average (up to 6 cm in hotspots), exacerbating relative sea-level rise. Concurrently, water quality is deteriorating in some stretches due to agrochemical runoff, industrial pollutants, and mining. Pollution, while beyond this paper’s main scope, intersects with health risks (e.g., unsafe drinking water leading to disease – see Section 2.4).
Energy Risks
Hydropower Development Challenges: Hydropower is a double-edged sword: it provides renewable electricity and revenue (particularly for Laos, dubbed the region’s “battery”), but it also introduces social-environmental trade-offs and risks of over-reliance. Extreme droughts have revealed hydropower’s vulnerability: during the 2019–2020 drought, Cambodia’s hydroelectric output fell ~30%, causing nationwide blackouts. With climate projections indicating more erratic rainfall and intensified El Niño events, future hydropower generation is insecure. If reservoir levels drop, electricity shortages ripple across economies. This risk is particularly acute for Cambodia, Laos, and Myanmar, which rely heavily on hydro.
Transboundary Energy Conflicts: Dams on a shared river can ignite geopolitical frictions. Upstream-downstream tensions have already surfaced. For instance, Laos’s Xayaburi Dam – the first lower mainstream dam – drew objections from Cambodia and Vietnam due to expected downstream impacts. The MRC’s prior consultation process, while a platform for dialogue, lacks binding enforcement. Consequently, unilateral decisions (like Laos moving ahead with dams despite neighbors’ concerns) risk trust deficits and diplomatic strains. Downstream, reduced fish stocks and altered flows (attributed to upstream dams) sometimes feed resentment and even conspiracy narratives during crises (e.g., blaming Chinese dams for droughts). Energy security interdependence is also emerging: Thailand and Vietnam buy power from Lao dams, creating complex incentives. A key risk is overbuilding – Laos’s aggressive dam rollout could lead to a power glut and debt burden (stranded assets) if neighbors diversify away from imports. This misalignment of national plans is a governance challenge and an economic risk (see Section 3).
Alternative Energy Sources: The flip side of hydropower risk is the rising feasibility of alternative energy – particularly solar and wind – which can reduce the need for new dams. Vietnam has demonstrated a solar boom: propelled by feed-in tariffs, it added over 12 GW of solar capacity from 2019 to 2021, leading Southeast Asia. By 2024, Vietnam had ~19.5 GW of utility-scale solar and wind installed, accounting for 25% of its power mix. This rapid expansion showcases how policy incentives and falling technology costs can reshape the energy landscape. However, integrating variable renewables without robust grids and storage is a new challenge, as Vietnam experienced curtailment issues (excess solar wasted due to grid limits). For the Mekong region, solar and wind offer a chance to balance the energy mix and alleviate pressure on the river (potentially displacing some planned hydropower). Yet, without regional coordination (e.g., power trade agreements, grid interconnections), countries might not fully capitalize on these alternatives. In summary, energy risks revolve around balancing hydropower’s benefits against its vulnerabilities and externalities, managing transboundary tensions it creates, and seizing new opportunities in renewables to build a more resilient energy system.
Food Security Risks
Agricultural Expansion and Intensification: The Mekong Basin’s fertile plains support tens of millions of farmers. It’s a rice powerhouse – the Mekong Delta alone produces over half of Vietnam’s rice and 70% of its fruit and 60% of seafood exports. Expansion of agriculture (often at the expense of forests and wetlands) has boosted food output but introduced dependencies on controlled water and agrochemicals. Intensive triple-cropping in the delta, for instance, requires heavy irrigation and has stopped natural sediment deposition (because floodwaters are kept out by dikes). This undermines soil health over time and increases fertilizer needs. Additionally, conversion of floodplain forests and wetlands reduces wild fish nurseries and soil retention, potentially decreasing long-term productivity.
Fisheries Depletion: Wild-capture fisheries in the Mekong are critical for diets and incomes, especially in Cambodia and Laos. The Mekong is the world’s largest inland fishery, yielding 15% of global inland catch (~2.3 million tonnes annually) and feeding ~40 million people. This fishery is under grave threat. Dams fragment habitats and block fish migrations, directly reducing breeding success for migratory species. An MRC study predicted Mekong dam development could deplete fish biomass by 40% by 2020 and 80% by 2040. Particularly at risk is the Tonle Sap Lake – the “beating heart” of the Mekong – where altered flows and trapped sediment from upstream dams have led to shorter, smaller floods, jeopardizing the nursery ground for many fish species. Overfishing and illegal fishing (using fine nets, electrofishing) compound the issue, as human populations have grown and fish catch becomes more commercialized. The loss of fisheries has direct food security implications: for example, ~80% of Cambodians’ protein intake traditionally comes from Mekong fish. If fish catches plummet, communities will face nutrition shortfalls and economic hardship unless alternative livelihoods or protein sources are available.
Impacts of Environmental Degradation: Environmental degradation – from deforestation to pollution – loops back into food security. Deforestation in upstream watersheds can cause more erratic river flows and sedimentation patterns, affecting downstream farm systems. Sand mining in the riverbed is another factor: rampant sand extraction (for construction) in the delta is “starving” the river of sediment, contributing to riverbank collapse. Bank erosion can swallow farmland (as with Vietnamese farmers watching fields slump into the river) and force relocations. Soil fertility is at risk too – without annual sediment deposits, delta soils degrade. Salinity intrusion is a growing threat in coastal farmlands: as the Mekong’s dry-season flow dwindles and seas rise, saltwater pushes further inland, damaging rice paddies. In 2019–2020, salinity intrusion in the Mekong Delta reached record levels, affecting 10 of 13 provinces and damaging tens of thousands of hectares of rice and fruit trees. Some projections suggest ~10% of the delta’s dry-season rice area could be lost by 2050 due to salinity, even under moderate climate scenarios. Food security risks, therefore, span immediate shocks (droughts or floods destroying crops, sudden fish declines) and gradual declines (soil fertility loss, cumulative ecosystem degradation), all of which threaten the reliability and quantity of food production in the basin.
Health Risks
Waterborne Diseases: Water is intimately tied to health in the Mekong Basin. Inadequate access to clean water and sanitation remains a challenge in parts of the region – for instance, millions in the Mekong Delta lack safe drinking water, contributing to diarrheal diseases. Stagnant or polluted waters (common during floods or in poorly managed canals) can breed cholera, dysentery, and other waterborne pathogens. The Mekong’s floods, while beneficial for ecosystems, often contaminate drinking sources, leading to spikes in gastrointestinal illnesses. According to the WHO, waterborne diseases and poor sanitation are leading causes of child mortality globally; the Mekong countries are no exception. Climate change exacerbates this risk: higher temperatures and more intense rain can increase bacterial growth and runoff of waste into waterways. A study noted that with rising temperatures, the incidence of diarrheal diseases could climb, as seen in Vietnam’s Mekong Delta where drought and saltwater intrusion in 2019–2020 left over 100,000 households short of clean water, elevating hygiene-related health risks.
Vector-borne Illnesses: The basin’s warm, humid climate is ideal for mosquitoes and other vectors. Dengue fever is a recurrent threat in the Mekong region, with large outbreaks occurring cyclically. Climate trends (warmer temperatures, altered rainfall) are expanding mosquito habitats and possibly lengthening transmission seasons. The WHO flags mosquito-borne diseases (malaria, dengue) as priority health concerns in the Lower Mekong, given how climate change could transform these diseases “into health emergencies”. For example, unusual rainfall patterns can create new breeding sites, and warmer weather speeds up mosquito reproduction and virus replication, raising transmission rates. Malaria control efforts have made progress, but border areas and forest fringes still see cases, and drug-resistant malaria strains in the Mekong region pose global concerns. Additionally, zoonotic diseases (transmitted from animals to humans) are a worry as deforestation and wildlife trade in the region heighten exposure to novel pathogens.
Environmental Change and Human Health: Beyond infectious diseases, environmental changes in the Mekong are affecting nutrition and livelihoods, which are key determinants of health. Fisheries decline, as discussed, can lead to protein and micronutrient deficiencies (fish is a major source of protein and vitamins for rural communities). Undernutrition is already an issue among marginalized groups; climate change threatens to worsen it by disrupting agriculture and fisheries. The Lower Mekong is highlighted as especially vulnerable to undernutrition linked to climate shifts, which could precipitate food insecurity and stunting in children. Extreme weather events (floods, storms) also cause injury, displacement, and mental health stress. For instance, flash floods can lead to drownings and trauma, while prolonged droughts contribute to economic stress and even conflict over water – indirectly affecting community well-being and mental health.
Health Systems and Compounding Stressors: A critical aspect of health risk is the capacity of health systems to respond. Many Mekong communities are rural with limited healthcare access. Poverty and inequality mean that those most exposed (e.g., subsistence farmers, fishermen) have the least resources to cope or seek treatment. Climate change can strain these health systems further; e.g., simultaneous dengue outbreaks and flood relief operations can overwhelm local clinics. As one report put it, without adaptation, disease outbreaks could “exceed the capacity to respond,” especially where poverty and weak health infrastructure prevail. Thus, the nexus of water-food security and health is evident: droughts and crop failures increase undernutrition, which in turn heightens vulnerability to disease; floods and poor water cause disease, which undermine productivity and food security – a vicious cycle if not addressed holistically.
Climate Risks
Extreme Weather Events: The Mekong Basin is experiencing more extreme weather. In recent years, record-breaking droughts (e.g., 2015–2016, 2019–2020) and floods have occurred with alarming frequency. Droughts reduce river flow to unprecedented lows – 2019 saw the lowest Mekong levels in over a century in some reaches. This led to emergencies in Thailand and Cambodia and exposed limitations in existing water-sharing arrangements (China released some water from its dams in 2020 to alleviate downstream drought, but questions remain on coordination). Floods, conversely, have become flashier: intense rainfall causes rapid inundation. The annual monsoon flood regime is inherently expected, but urban growth and deforestation have increased flood damage (less natural absorption, more assets in harm’s way). For example, severe floods in 2011 and 2013 affected millions in Thailand and Cambodia, causing significant economic losses.
Rising Temperatures and Heatwaves: Mean temperatures in the basin are climbing. By 2050, parts of the region could see average increases of ~2 °C (and up to ~4 °C by 2100 under high emission scenarios). Higher temperatures intensify evapotranspiration, stressing crops and water supplies. They also heighten heat stress on laborers – a notable issue as a large share of the population works outdoors in farming, construction, etc. Prolonged heat waves can impact public health (heatstroke, cardiovascular problems) and reduce agricultural yields (heat can damage rice flowers or stress livestock).
Shifting Monsoon Patterns: The onset, duration, and intensity of the Southeast Asian monsoon are changing. There are indications of delays in rainy season onset and more erratic intra-seasonal breaks. Farmers, traditionally attuned to predictable seasonal calendars, now face uncertainty: planting too early or late carries risk if rains don’t align. This can reduce crop yields and increase the need for irrigation (putting more pressure on water resources). Moreover, if rains become more concentrated, the risk of storm-induced flooding grows. Tropical storms that track into the Mekong (from the South China Sea) may become fewer but stronger, according to some climate studies, which would elevate disaster risks episodically.
Sea-Level Rise and Salinity: Though often considered a coastal issue, sea-level rise is a direct climate risk for the Mekong Delta – a low-lying area <2 meters above sea level on average. Projections suggest that with just 50 cm of effective sea-level rise (including subsidence), ~30% of the delta’s area could be below mean sea level. The delta is sinking due to sediment loss and groundwater pumping, and the sea is encroaching. Saltwater intrusion, as already noted, is expected to worsen, potentially rendering large tracts of land uncultivable or forcing shifts to salt-tolerant crops and brackish aquaculture. In essence, climate change poses compound risks: it can simultaneously reduce water availability (via drought), increase water excess (via floods), degrade water quality (via salinity), threaten food production, and spur health crises – all of which are interrelated under the WEFH nexus.
Governance and Policy Challenges
Transboundary Water Governance: Managing the Mekong’s risks requires cooperation among riparian countries – a formidable challenge. The Mekong River Commission (MRC), comprising Cambodia, Laos, Thailand, and Vietnam (with China and Myanmar as dialogue partners), is the chief mechanism for basin governance. The 1995 Mekong Agreement espouses “reasonable and equitable use” and mandates prior consultation for projects like dams. However, the MRC lacks enforcement power; its decisions are non-binding. This has been tested by mainstream dam projects (e.g., Xayaburi and Don Sahong in Laos) where procedural compliance occurred, but Laos proceeded despite downstream objections. China’s absence as a member is a major limitation – with China controlling the Lancang headwaters, it wields significant upstream influence yet is not bound by MRC protocols. In recent years, China has engaged via the Lancang-Mekong Cooperation (LMC) framework (launched 2016), promoting itself as a partner in water data-sharing and offering drought relief releases. While this is a positive step, the LMC is led by China and might parallel or even compete with the MRC, raising questions about coherence.
Regional Cooperation Mechanisms: Beyond the MRC and LMC, other bodies and agreements influence the WEFH nexus governance. ASEAN, though broadly focused, provides a platform for dialogues on sustainability and disaster management. The GMS Economic Cooperation Program (under ADB auspices) has advanced cross-border infrastructure and trade – beneficial for development but sometimes at odds with environmental stewardship. For instance, GMS investment in roads and power lines facilitated hydropower expansion and land use change without robust environmental safeguards. Policy misalignment among countries is evident: Laos prioritizes hydropower exports for revenue, Cambodia and Vietnam emphasize fisheries and delta protection, and Thailand pursues a mixed strategy (importing power but also investing in renewables). Aligning these interests is difficult. The 2010s saw failed attempts to establish a joint basin-wide impact assessment for mainstream dams; while some studies were done (e.g., the MRC Council Study), implementation of recommendations has lagged.
National Policies and Trade-offs: Each Mekong country faces internal policy challenges. Cambodia struggles to balance energy access with protecting fisheries – its 10-year dam moratorium until 2030 reflects a policy shift toward coal, gas, and solar to meet energy needs. This decision, applauded by environmentalists, may strain budgets (fossil fuel imports) but acknowledges the vital role of a healthy Mekong for agriculture and nutrition. Laos has embraced a dam-building agenda, often in partnership with foreign investors, aiming to graduate from Least Developed Country status by exporting electricity. However, concerns of debt traps and over-reliance on a single sector loom; a dam collapse in 2018 (Xe Pian-Xe Namnoy saddle dam failure) also raised questions about oversight and safety standards in Laos’s hydropower sector. Thailand largely halted domestic mainstream dams after public backlash (e.g., the Pak Mun dam controversy of the 1990s), but Thai companies and banks invest heavily in Lao projects, externalizing impacts. Thai water policy now grapples more with irrigation efficiency and flood control at home, including controversial diversion schemes for drought-prone northeast regions, which could affect transboundary flows.
Legal and Institutional Gaps: Internationally, the Mekong is not governed by a comprehensive treaty including all riparians. The 1997 UN Convention on non-navigational water uses (the global watercourses convention) is not ratified by most Mekong states. Hence, no overarching legal framework unequivocally mandates data sharing or joint management. This gap means downstream countries often resort to diplomacy or international pressure to influence upstream behavior, with mixed success. For example, during the 2019 drought, Vietnam repeatedly called on China to release more water; China responded but only after delays and under its own terms. The absence of China and Myanmar in the MRC and the non-binding nature of its processes (PNPCA – Procedures for Notification, Prior Consultation and Agreement) limit the MRC’s ability to proactively mitigate risk. It tends to function more as a technical advisory body, producing valuable analyses (e.g., fisheries reports, climate scenarios) but unable to enforce environmentally sound operation of dams or land-use practices.
Enforcement and Compliance Issues: Even within countries, enforcement of regulations is a challenge. Illegal fishing methods persist due to lax enforcement, undermining fisheries management. Protected area laws exist for critical habitats, but illegal logging and wildlife trade continue, reflecting governance weaknesses. The “commons” problem is acute: the Mekong’s water and fish are shared resources that require collective action, yet national interests often prevail. As Middleton (2022) and others argue, power asymmetries (e.g., China’s leverage, or elite capture of benefits) shape outcomes, sometimes sidelining local communities. Equitable governance – ensuring that dam-affected communities receive justice or that small fishers have a voice – is part of the challenge. Without improved governance, risk mitigation efforts (like coordinated dam operations for flood control, or joint disaster response plans) may fall short. Strengthening institutions like the MRC, expanding data sharing (China’s recent agreement to share year-round Lancang flow data is a start), and creating enforceable norms for sustainable river use are critical policy-front challenges moving forward.
Technological and Economic Trends
Infrastructure and Connectivity Booms: The Mekong region’s economy has grown rapidly, averaging 5-7% annual GDP growth in many Mekong countries pre-COVID. This growth is coupled with large infrastructure investments, often under China’s Belt and Road Initiative (BRI). Since 2013, Chinese-led investments have improved connectivity via railways, highways, power grids, and telecommunications networks. For example, the China–Laos railway (opened 2021) connects Kunming to Vientiane, fostering trade (and opening new corridors for agricultural exports and tourism). Special economic zones, like Cambodia’s Sihanoukville or the Lao-Thai border zones (e.g., Mohan–Boten SEZ), are attracting manufacturing and trade logistics. These developments indicate a structural shift: from subsistence agrarian economies to more industrialized, interconnected ones.
However, this transformation has resource implications. New highways can bisect ecosystems, rail connectivity can facilitate faster land-use change (e.g., access to remote forest areas), and economic zones need power and water – potentially increasing demand for hydropower or large-scale irrigation. On the positive side, better roads can mean easier disaster relief access and markets for farmers, improving resilience if managed well. Technology transfer, such as more efficient irrigation (drip systems, sensor-based water management) and climate-smart agriculture, is starting to take root via regional initiatives (e.g., ADB’s climate-resilient rice or the GMS Core Agriculture Support Program promoting agritech).
Innovations in Resource Management: Technological innovation is playing a growing role in addressing nexus challenges. For water, there’s increased use of remote sensing and modeling to predict floods/droughts and plan responses. For example, satellite data analyses by groups like SERVIR-Mekong help map flood extents and monitor reservoir levels in near real-time. In agriculture, drought-tolerant rice varieties and System of Rice Intensification (SRI) methods are being introduced to maintain yields with less water. The region is also seeing pilots in solar pumping for irrigation and floating solar farms on reservoirs, which exemplify cross-sector synergy (energy-water co-benefit). Additionally, the concept of the WEF nexus has spurred integrated projects: Thailand’s P-LINK Water-Energy-Food Nexus pilot (supported by UN and Korean partners) is implementing smart meters, reducing water leakage, and coupling biogas energy recovery with agricultural systems.
Economic Shifts and Trade: The Mekong economies are diversifying. Thailand and Vietnam are now middle-income with substantial manufacturing (electronics, garments) and service sectors. Cambodia and Laos remain more agrarian but are urbanizing. As economies shift, resource use patterns change. Diets diversify (potentially lowering per-capita rice consumption but increasing water-intensive meat demand), and energy needs shift from basic (cooking fuel) to industrial (electricity for factories, cooling for cities). Economic integration means that shocks transmit faster: a flood in Thailand can disrupt global supply chains (e.g., 2011 Thai floods hit hard-drive manufacturing worldwide), and a drought in China’s Yunnan can reduce power imports to Thailand, affecting industries.
Investment and Funding Trends: There is a notable trend toward “green” financing and sustainability in rhetoric, if not fully in practice. Multilateral development banks (World Bank, ADB) have become more cautious on funding Mekong mainstream dams due to environmental concerns, leading project sponsors to seek private or Chinese finance. Conversely, those banks are funding more climate adaptation projects: e.g., World Bank’s Mekong Integrated Water Resources Management or ADB’s flood management program in the Mekong Delta. Payment for ecosystem services (PES) and community-based conservation (like co-management of fisheries or community forestry) are emerging as win-win approaches, but require supportive policy and capacity.
Technology and Data for Resilience: A critical positive trend is the advancement of early warning systems. The MRC and national agencies have improved flood forecasting; the goal is to also build drought forecasting capacity. Mobile technology means warnings (flood crest predictions, dam release notices) can reach villages faster. Social media and community radio have been used in disaster communication. Moreover, the wealth of research and open data (such as the Mekong Data Portal, or Open Development Mekong platforms) allows civil society and academics to contribute to transparency and accountability, potentially leading to better governance outcomes.
In summary, technological and economic trends in the Mekong Basin are a mix of opportunities (for innovation, efficiency, and resilience) and new pressures (through rapid development and resource consumption). Harnessing these trends for risk mitigation – for instance, using regional power grid upgrades to integrate solar/wind and reduce hydropower dependence, or employing climate-smart agriculture to secure food production – is essential for a resilient future.
Critical Transitions and Inflection Points
Reflecting on the Mekong Basin’s trajectory, several critical transitions stand out as inflection points that have reshaped risk profiles:
Transition 1: Conflict to Cooperation (1950s–1990s) – The mid-20th century saw colonial and Cold War conflicts halting large-scale development. An inflection point came with the Paris Peace Accords (1991) and the reintegration of Cambodia, enabling basin-wide cooperation. The establishment of the MRC in 1995 signified a hopeful turn toward joint management. This transition laid the groundwork for all subsequent developments by providing a forum (albeit limited) for dialogue and planning.
Transition 2: Free-flowing to Dam-regulated River (1990s–2010s) – The aggressive pursuit of hydropower, particularly by China and Laos, marks a fundamental shift in the river’s nature. Inflection Point: Manwan Dam (China, 1993) – first on the mainstream – and Pak Mun Dam (Thailand, 1994) on a major tributary, which symbolized the new era of dam-building. A major tipping point was around 2010–2012, when Laos moved on Xayaburi despite regional pushback. By 2020, with multiple mainstream dams operational, the Mekong’s flow and ecology had irreversibly changed. This transition increased water regulation capacity (theoretically helping dry-season flows) but simultaneously introduced downstream sediment starvation and fishery fragmentation as chronic issues. It also shifted power dynamics – upstream countries gained leverage through control of dams.
Transition 3: Agrarian to Mixed Economies (2000s–present) – Over the last two decades, Mekong countries have experienced rapid economic growth and structural shifts. A key inflection point was Vietnam’s Đổi Mới (economic reforms of the late 1980s) and Cambodia’s similar opening in the 1990s, which propelled integration into global markets. The proliferation of BRI projects post-2013 further accelerated infrastructure development. This transition lifted millions out of poverty but also increased resource extraction (e.g., rubber and palm oil booms in Cambodia/Laos), urban pollution, and demand for energy and water. It’s a story of rising living standards accompanied by environmental stress – a classic development-environment trade-off that the region is still navigating.
Transition 4: Environmental Awakening and Policy Shift (mid-2010s–present) – Signs of an environmental inflection have appeared as the impacts of the above changes manifest. 2015-2016 Drought & Mekong Delta Crisis was a wake-up call – the worst drought in a century, compounded by El Niño and upstream dam operations, caused saline intrusion deep into the delta, crop failures, and rural exodus. This crisis, plus scientific studies (e.g., MRC’s Council Study, WWF reports), started to influence policy. In 2020, Cambodia’s dam moratorium was a landmark decision acknowledging that immediate energy gains were not worth the long-term food security and livelihood losses. Thailand’s continued refusal to buy power from new Mekong mainstream dams (favoring cheaper solar/wind) is another sign of shifting calculus. Civil society’s role grew too – transboundary campaigns and local protests highlighted social costs of projects (like the displacement from dams, or sand mining erosion). The narrative is slowly transitioning from “economic growth at any cost” to “sustainable development and resilience.” Still, implementation is nascent; the inflection is incomplete but noteworthy.
Transition 5: Climate Adaptation Imperative (emerging) – Finally, an incipient transition is the region bracing for climate change. After years of mainly reactive disaster response, countries are starting to incorporate adaptation into planning. Vietnam’s government, for example, approved a Mekong Delta Plan focusing on “living with water” (accepting more floods, shifting from rice to aquaculture in salinized zones) – a strategic pivot from trying to control nature to adapting to it. The Greater Mekong Subregion is investing in climate-smart infrastructure (like flood-resilient roads, improved drainage in cities). The inflection point here might be now: as climate impacts intensify, policies and investments over the next decade will determine if the basin can transition to a climate-resilient pathway or if it will suffer escalating damages.
Each of these transitions interlinks with WEFH nexus elements: cooperation allowed broader planning (Water), dam-regulation affected energy and food (Energy-Food), economic shifts altered consumption and health patterns (Food-Health), environmental awakening is about balancing all nexus elements, and climate adaptation touches water, food, health concurrently. Recognizing these inflection points helps target where interventions and resilience strategies (discussed next) can be most impactful.
The case of The Mississippi River Basin
Nour Atrakouti
Introduction
There are two cities around the world that share the name Cairo: one on the banks of the Nile, a river that has shaped the history and development of North Africa, and another, Cairo, Illinois, located at the confluence of the Mississippi and Ohio Rivers. Like the Nile, the Mississippi River has served as a lifeline, supporting economic, agricultural, and energy systems across North America. Stretching approximately 3,781 km across the United States, “the Mississippi River watershed, the largest in the United States (41% of the area of the contiguous 48 states), has undergone massive transformations in the last 200 years as it has developed into what Samuel Clemens described as ‘the body of the nation’ and others have called the nation’s ‘breadbasket’” (Turner & Rabalais, 1991).
The Mississippi River has played an integral role in shaping the history of the United States. “Prior to the Louisiana Purchase, the Mississippi River acted as the western border for the United States. The waterway was first used for trade with Indian tribes when fur pelts were floated down the river from Ohio. Once steamboats were invented, the Mississippi River became an important mode of transportation that revolutionized river commerce” (U.S. Environmental Protection Agency [EPA], 2025). Human interventions, including the construction of locks and dams, were implemented over time to manage flooding and deepen waterways for steamboats. These modifications, along with large-scale agricultural expansion, urbanization, and industrialization, have profoundly altered the river’s hydrology and ecological health.
Governance of the Mississippi River basin has evolved in response to shifting priorities, from navigation and agricultural development to disaster management and climate adaptation. However, “managing this complex basin has been an intricate undertaking, shaped by varying needs and priorities across different periods and communities” (Guo, 2023). Reactions to natural disasters, in particular, have differed throughout history, influencing long-term strategies for sustaining this essential resource. This report will examine the historical evolution of the basin through a trend analysis approach, identifying key transitions in water management, agriculture, infrastructure, and environmental policy. These insights will provide essential historical context for addressing contemporary challenges and guiding future interventions.
Further supporting Chapter 3, on Interlinkages and Cascading Effects, this chapter and case study further demonstrate the importance of studying and analyzing the different components of the Nexus simultaneously, in order to measure spill-over effects and understand the causal relationships that often link these components. Understanding these relationships, coupled with a temporal view of a natural resource’s history and characteristics, allows for accurate and transformative policy recommendations. These recommendations, whose impact can be traced and measured in the future, also help outline guidelines for policy implementation, learning from past mistakes and adapting accordingly.
→ Terminology Disclaimer:
Throughout this report, the term ‘Gulf of Mexico’ is used to refer to the body of water historically known by this name. While it has recently undergone a formal name change, this report retains the original designation for consistency and alignment with the terminology used in multiple official sources and archival records, including but not limited to documents from the United States Environmental Protection Agency (EPA).
Resources of the Mississippi River Basin:
The Mississippi River Basin is a critical natural resource, supporting agriculture, transportation, water supply, and biodiversity:
Food and Transportation
Agriculture is a major economic driver in the basin, with approximately 60% of the land used for farming (Guo, 2023). The central part of the basin, known as the “Corn Belt,” is a key area for corn and soybean production, which are essential to both national and global food markets: “This region is known as the ‘Corn Belt,’ but today it produces large amounts of both corn and soybeans” (Guo, 2023). Beyond agriculture, the river system is crucial for transportation. The Mississippi provides substantial cost savings for moving goods. “Annually, the regional project generates an estimated $3 billion of transportation cost savings compared to overland methods” (U.S. Army Corps of Engineers, 2024). The urgency of addressing the Mississippi River’s challenges is amplified by its essential role as a lifeline, sustaining both the agricultural heartland and vital transportation networks that countless communities, across states, depend on.
Water and Industry
The Mississippi River Basin drains 41% of the contiguous U.S., making it a critical water resource. “If we have clean water, then our economy has a competitive edge in that it too is sustainable. Most importantly, if we have clean water, then the 50 cities (20 million people) drinking the water can be assured of a secure source into the future” (Mississippi River Cities and Towns Initiative, 2016). The river also supports significant industrial activity, with manufacturing using vast quantities of water. “The River Corridor is one of the most intensive surface water use regions, withdrawing a minimum of 38 billion gallons annually” (Mississippi River Cities and Towns Initiative, 2016). This reliance on water for both agriculture and industry highlights the importance of clean water for economic stability and public health.
Biodiversity and Ecology
The basin is also a vital ecosystem, supporting a rich diversity of wildlife. “Sixty percent of North American birds use the Mississippi River as their migratory flyway” (U.S. Environmental Protection Agency [EPA], no date), and the river supports a wide range of fish and invertebrates, including several endangered species like the pallid sturgeon. Additionally, the river’s ecology drives economic activity through recreation, with the Upper Mississippi River’s recreational impacts estimated at over $1 billion annually (National Research Council et al., 2008).
Historical Challenges and Significant Events in the Mississippi River Basin
Agriculture and Food Security
Agricultural activities in the Mississippi River Basin have played a significant role in shaping the region’s environmental and socio-economic landscape. A study exploring changes in water quality in the Mississippi River Basin throughout the 20th century concluded that “water quality in the river has most certainly changed as a result of these watershed changes and changes in fertilizer use. [As its use increases]” (Turner & Rabalais, 1991). The increased use of nitrogen and phosphorus fertilizers has had a significant impact on biodiversity and water quality, leading to widespread nutrient pollution. And it can be concluded that “agriculture contributes the major portion of nutrients and sediments delivered to the Mississippi River” (The National Academy of Sciences, 2007). Having a chance at improving water quality in the Mississippi River is ensuring that policies focusing on the matter prioritize and push for an urgent and rapid reduction in nutrient pollutants, with the agricultural sector as a central target for these effort.
The U.S. Department of Agriculture (USDA) has administered “several incentive-based conservation programs designed to implement best management practices (BMPs) to reduce levels of nutrient and sediment in runoff” The National Academy of Sciences, 2007). Although participation in these programs is voluntary, the potential for financial gain offers these programs strong incentives for adoption. Addressing water quality issues effectively, relies heavily on adopting management strategies that consider the unique characteristics of each site: Effective management of nutrient and sediment inputs from agricultural sources requires approaches that are tailored to specific sites, focusing on areas with higher runoff The National Academy of Sciences, 2007). These targeted interventions are crucial in minimizing the impacts of agricultural runoff on water quality. Moreover, recent developments in biofuel production have led to an increase in nutrient and sediment pollutant loads, which compounds existing environmental concerns The National Academy of Sciences, 2007). This highlights the urgency of adopting localized strategies that can address the growing pressures on water resources, ensuring more sustainable agricultural practices.
Water Management and Quality
The Mississippi River has faced significant water management challenges due to industrialization, agricultural intensification, and infrastructural development. Across time, Increased river traffic has led to serious environmental impacts like bank erosion, heightened turbidity, and habitat disruption for native species, as “the increased amount of river dredging, levee building, and construction that comes along with this traffic impairs aquatic life in many ways by disturbing their habitat” (U.S. Environmental Protection Agency [EPA], 2025).
Nutrient pollution has emerged as a major issue in the Mississippi River watershed. “Today, however, the watershed of the Mississippi River suffers from the effects of excess inputs of nitrogen and phosphorus, often referred to as “nutrient pollution” [which is linked] to the contamination of drinking water, surface water, and groundwater throughout the Mississippi River watershed and to the growth of the hypoxic (oxygen-poor) zone in the northern Gulf of Mexico” (U.S. Environmental Protection Agency [EPA], no date). On the national level, 39% of streams in the Mississippi River Basin have high levels of nitrogen, and 32% have high levels of phosphorus, compared to the national averages of 32% and 31%, respectively (U.S. Environmental Protection Agency [EPA], 1972). These figures underscore the severity of nutrient pollution in the region, highlighting both its environmental impact and the risks it poses to public health. Trace organic pollutants in the Mississippi River’s drinking water supply raise significant health concerns, particularly for the 1.5 million people who depend on it , with vulnerable populations such as the elderly, the sick, and children being at the greatest risk (U.S. Environmental Protection Agency [EPA], 1972).
Human intervention has drastically altered the natural dynamics of the Mississippi River, with “the historical sediment load of the Mississippi River has been reduced by over 50% (Meade, 1995; Smith and Winkley, 1996), leading to the rapid subsidence of coastal marsh ecosystems” (DuBowy, 2013). This sediment reduction has profound implications for the ecological stability and resilience of these vital habitats. Additionally, the Mississippi River system has been overwhelmed by a rapid growth in non-native species (DuBowy, 2013), further disrupting native biodiversity and ecosystem balance.
Natural Disasters and Climate Impact:
Flooding and natural disasters have been recurring challenges for the Mississippi River Basin, as “discharge records from 66 stations on the Mississippi River system confirm a pattern of increasing flows” (Pinter et al., 2008), contributing to increases in total flood levels documented across the basin. This increased flood frequency and intensity exacerbate the region’s vulnerability to natural disasters.
Biodiversity in the Mississippi River Basin has also been threatened by industrial pollution and plastic waste. “The Mississippi River drains into the Gulf of Mexico, nourishing the Gulf’s important fisheries. However, along with the freshwater and sediment that the river carries, enormous amounts of plastic pollution flow from communities along the Mississippi River and its tributaries into the Gulf” (EPA Trash Free Waters, 2021). Plastic pollution poses long-term environmental risks, as it persists in the environment for centuries, creating hazards for wildlife and human populations. Microplastics, “including pre-production plastic pellets known as nurdles”, are particularly concerning due to their widespread presence and the difficulty of cleanup efforts (EPA Trash Free Waters, 2021).
Industrial Impact
Industrialization has left a lasting impact on the Mississippi River’s water quality and ecosystem health, with “many of the original freshwater wetlands, riparian zones, and adjacent streams and tributaries along the Mississippi […] disconnected from the river by levees and other engineering modifications” (U.S. Environmental Protection Agency [EPA], 2025). These alterations have disrupted natural water flow, reduced habitat connectivity, and diminished ecological resilience.
Going back to 1972, industrial waste discharges were identified as principal causes of persistent pollution issues: “The industrial waste discharges are the principal cause of the persistent ‘oily-petrochemical’ odor in the public water supplies downstream from Baton Rouge [with the] industrial waste discharges [being] the principal cause of the tainted flesh of fish caught in the Mississippi River downriver” (U.S. Environmental Protection Agency [EPA], 1972). This contamination not only affected the environment but also raised significant public health concerns. The presence of trace organics in the river’s drinking water posed “a potential threat to the health of the 1.5 million people who consume this water, particularly the elderly, those that are ill, and children” (U.S. Environmental Protection Agency [EPA], 1972).
In conclusion, the historical challenges and significant events outlined above illustrate the complex interplay between agriculture, water management, climate impact, and industrial development in the Mississippi River Basin. Understanding these historical trends and inflection points is essential for developing effective policy interventions and sustainable resource management strategies.
Policy and Governance History of the Mississippi River: A Chronological Overview:
The governance of the Mississippi River has evolved significantly, driven by growing environmental concerns, scientific research, and shifting political landscapes. In 1964, early policy recommendations highlighted the importance of controlling pollution from industrial and municipal waste. A key report noted, “sewage and industrial wastes discharged to the Mississippi River from Minnesota cause pollution that adversely affects the water quality” (U.S. Department of the Interior, 1964). The document emphasized the need to reduce waste discharge, signaling early recognition of the river’s role in public health and the economy.
The passage of the Clean Water Act (CWA) in 1972 marked a turning point, setting national goals to reduce pollutant discharges and improve water quality. The CWA sought to “achieve zero discharges of pollutants into navigable waters by 1985” and ensure that waters were “fishable and swimmable” by the mid-1980s The National Academy of Sciences, 2007). This legislation laid the foundation for stricter pollution control, addressing both point-source pollution and broader environmental health concerns in the Mississippi River.
By the 1990s, the issue of hypoxia, caused by nutrient runoff from the Mississippi River, became a growing concern. The EPA’s Hypoxia Task Force (HTF), established in 1997, aimed to “reduce the size, severity, and duration of the hypoxic zone and ameliorate the effects of hypoxia” by improving nutrient management (U.S. Environmental Protection Agency [EPA] Hypoxia Task Force, 2025). This shift moved from addressing point-source pollution to managing agricultural runoff, particularly nitrogen and phosphorus. In 2004, the HTF published a Science Strategy, which stressed the importance of “improving water-quality conditions in the Mississippi River Basin, reducing hypoxia in the northern Gulf of America, and improving the communities and economic conditions” (U.S. Environmental Protection Agency [EPA] Hypoxia Task Force, 2025). The strategy’s goal was to reduce nutrient pollution to protect the region from the growing environmental threat.
A 2007 EPA report, which aimed to evaluate the characteristics of the reasons behind the hypoxic zone in the Northern Gulf of America, confirmed the need for “management and control options in the Mississippi River Basin and Gulf to reduce [the hypoxic zone” (U.S. Environmental Protection Agency [EPA] Hypoxia Task Force, 2025). The emphasis being on practical measures to control agricultural runoff. In 2008, the HTF updated its Action Plan, recognizing the importance of “[acknowledging] the social, political, and economic changes links to emerging issues and policies” while reducing nutrient pollution (U.S. Environmental Protection Agency [EPA], 2008). The Task Force stressed the need for collaboration and “stakeholders to identify the appropriate funding strategies that will be the most effective in ensuring timely implementation to achieve measurable and effective results” (U.S. Environmental Protection Agency [EPA], 2008). This approach highlighted the complexity of managing the Mississippi River’s health, requiring both local and regional cooperation.
Recent efforts have continued to emphasize the importance of monitoring nutrient levels. A 2019 EPA grant of $1.2 million to several states in the Mississippi River Basin supported programs to reduce nutrient pollution (U.S. Environmental Protection Agency [EPA] Hypoxia Task Force, 2025). These grants focused on improving agricultural practices, enhancing wetland restoration, and better managing runoff to reduce the river’s nutrient load. By 2021, the HTF focused on the need for “track loads and trends in large rivers to help states evaluate progress toward meeting nutrient reduction goals and to support adaptive management of nutrient reduction strategies” (U.S. Environmental Protection Agency [EPA] Hypoxia Task Force, 2025). The Task Force emphasized data-driven approaches to decision-making, ensuring that policies were informed by real-time scientific research and monitoring. Flood management, an ongoing challenge for the Mississippi River, became central to U.S. policy following the catastrophic 1927 flood. The Flood Control Act of 1928 gave “the U.S. Army Corps of Engineers authority to oversee flood control”, through levees and dams (Guo, 2023). Over time, the Corps incorporated more sophisticated forecasting methods and floodplain zoning techniques. In recent decades, however, there has been a growing push for “the integration of nonstructural approaches, such as floodplain zoning and flood forecasting systems,” to complement traditional flood control infrastructure (Guo, 2023).
In the 21st century, climate change has increasingly influenced Mississippi River governance. The Mississippi River Basin Healthy Watersheds Initiative and other projects were launched by the USDA in 2023 “focusing on multiple objectives, such as flood control, water quality, habitat restoration, and pollution reduction” (Guo, 2023). Collaborative efforts with global organizations, such as the United Nations Environment Programme, have also focused on issues like plastic pollution in the river, urging the public to adopt sustainable practices The National Academy of Sciences, 2007).
In conclusion, the governance of the Mississippi River has transitioned from addressing basic pollution concerns to implementing comprehensive strategies for nutrient management, flood control, and ecosystem preservation. Through continued monitoring, collaboration, and policy evolution, efforts to protect this vital waterway continue to adapt to new challenges, ensuring its sustainability for future generations.
Key Takeways:
- Past to Present Transformation: The basin has changed drastically in the last 200 years due to agriculture, urbanization, and industry.
- Agriculture’s Impact: Increased fertilizer use in the 20th century worsened water quality. Agriculture is a major polluter through nutrient and sediment runoff.
- River Modifications: Locks, dams, and levees altered the river’s flow, reducing sediment and impacting coastal ecosystems.
- Evolving Governance: Management shifted from focusing on navigation and agriculture to addressing disasters and climate change.
- Industrial Pollution: Industrial waste has been a persistent source of water pollution.
- Flooding: Increased river flows are leading to more frequent and intense flooding.
- Need for Targeted Solutions: Effective water quality management requires strategies tailored to specific locations.
- Urgent Action Needed: Rising biofuel production increases pressure on water resources, requiring immediate action.
The Case of The Amazon Basin
Francis Mwakina
Executive Summary
This report examines the intricate relationships among water, food, energy, health, and climate within the Amazon Basin in Brazil, emphasizing the importance of understanding historical contexts and trends. By analyzing historical drivers, trend shifts, and path dependencies, this study aims to identify critical transitions and inflection points that have shaped the region’s socio-ecological dynamics.
Utilizing a comprehensive range of data sources, including reports from international organizations and scholarly articles from peer-reviewed journals, this analysis provides a robust foundation for scenario modeling. The insights gained will inform future interventions, enabling policymakers and stakeholders to make informed decisions that promote sustainability and resilience in the face of ongoing environmental changes.
Introduction
1.1. Background
The Amazon Basin, often referred to as the “lungs of the Earth,” is a region of immense ecological significance and complexity, covering approximately 6.1 million square kilometers (Murtoff, 2025).
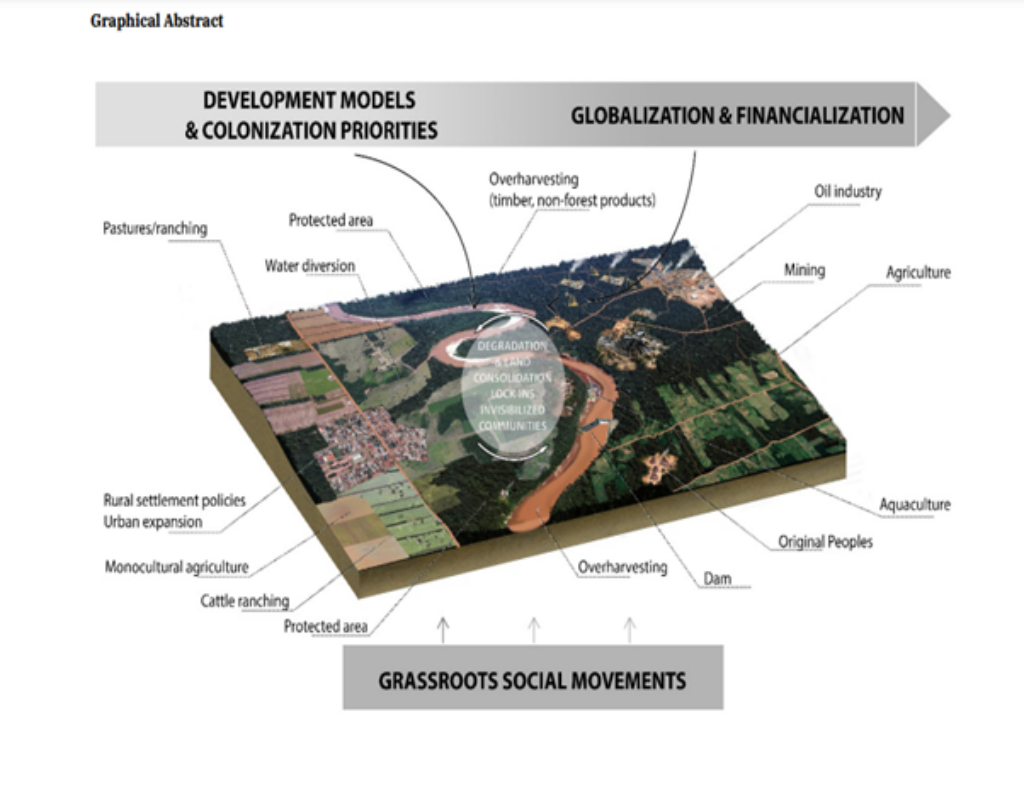
Figure 1: Amazonian Landscape
The choice to focus on the Amazon Basin for this trend analysis stems from its critical role in global environmental systems and its unique socio-ecological dynamics. Amazon has been in motion since the 19 century due to the critical transitions and trends happening within the basin from the countries within the basin region.
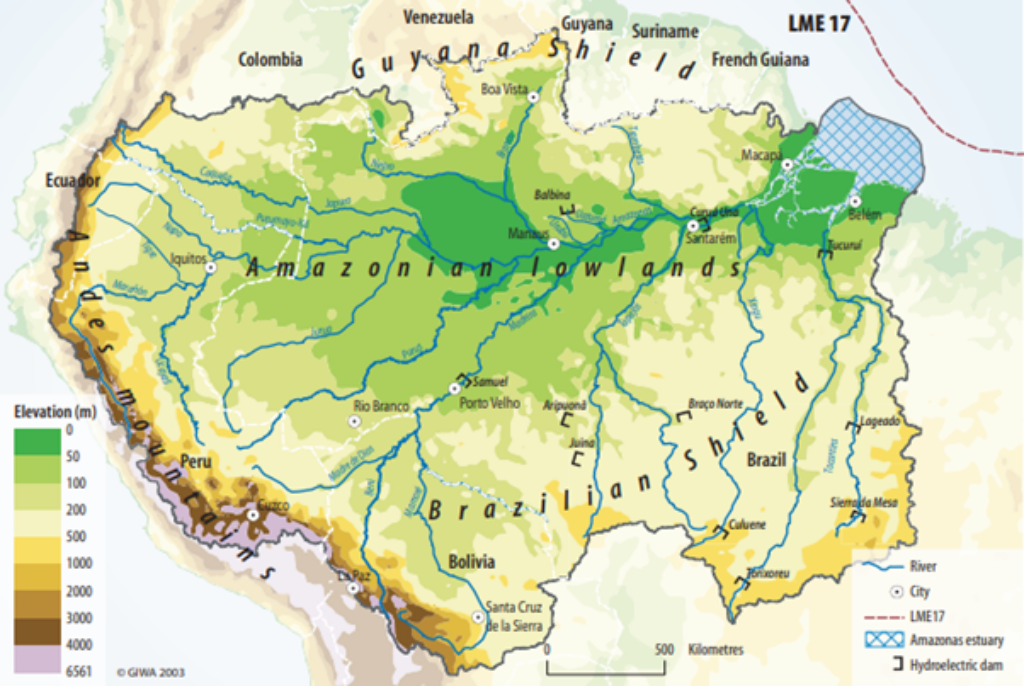
Figure 2: The Amazon Basin
As the region grapples with the challenges posed by deforestation, climate change, and socio-economic pressures, understanding the historical context and trends becomes essential for effective intervention and policy-making (CDKN, 2016)
Historically, the Amazon has been shaped by a multitude of factors, including colonization, agricultural expansion, and industrialization. These historical drivers have not only influenced the ecological balance but have also impacted the livelihoods of local communities. By examining these historical contexts, we can uncover the underlying patterns that have led to current environmental issues and identify potential pathways for sustainable development. The analysis will also highlight critical transitions, such as shifts in land use and resource management practices, that have significant implications for water, food, energy, and health within the basin (Environmental Encyclopedia, 2025)
1.2. Scope of Influence
The water-energy-food-health-climate nexus is an essential framework for examining the interrelations of vital resources in the Amazon Basin, highlighting the effects of historical factors like deforestation and agricultural expansion on socio-ecological systems. The Amazon Basin in Brazil is a significant area of study because Brazil encompasses the largest portion of the Amazon rainforest.
Table 1: Countries within the Amazon Basin
Country | Amazon Basin by country (%) | Country area included in the Amazon Basin (%) |
Brazil | 69.1 | 54.7 |
Peru | 11.4 | 59.9 |
Bolivia | 10.7 | 65.9 |
Colombia | 5.9 | 35.0 |
Ecuador | 2.0 | 46.8 |
Venezuela | 0.8 | 6.1 |
Guyana | < 0.1 | <0.1 |
Source: (UNEP 2004)
This framework facilitates the understanding of trends in water availability, energy production, food security, and health, while also supporting the United Nations Sustainable Development Goals.
The Amazon Basin has been influenced by various historical drivers such as deforestation, agricultural expansion, and climate change. These factors have altered the basin’s hydrology, energy dynamics, and food systems. For instance, the expansion of agriculture, driven by both local and global demand for commodities like soy and beef, has led to significant deforestation, which in turn affects water cycles and biodiversity.Climate change increases risks to forest stability, with studies identifying critical “tipping points” of 4° C warming or 40% deforestation that should not be exceeded (Nobre, 2016)
1.3. Research Objectives
This report aims to analyze the intricate interconnections among water, food, energy, health, and climate within the Amazon Basin, highlighting how these elements influence one another. It will explore the historical drivers and trends that have shaped socio-ecological dynamics, identifying critical transitions and inflection points that significantly impact sustainability. By developing a comprehensive understanding of the current state of resources and their interrelations, the report will provide actionable recommendations for policymakers and stakeholders. These recommendations will focus on enhancing sustainability and resilience in the Amazon Basin, addressing the ongoing environmental changes and challenges the region faces. This analysis will serve as a foundational basis for scenario modeling and future interventions aimed at promoting a sustainable future for the Amazon Basin.
Historical Context of the Amazon Basin
2.1. Resource Availability
Over the years, the Amazon has experienced significant changes due to both natural and anthropogenic factors, leading to shifts in resource distribution and availability. Historically, the region has been rich in biodiversity and natural resources, supporting indigenous communities and diverse ecosystems. However, increasing deforestation, agricultural expansion, and climate change have altered these dynamics, impacting water availability, food security, energy production, and health outcomes.
The interplay between these elements has become increasingly complex, as challenges in one area can exacerbate issues in another. Forest clearing in Brazil increased by almost 66% from 2013 to 2014.
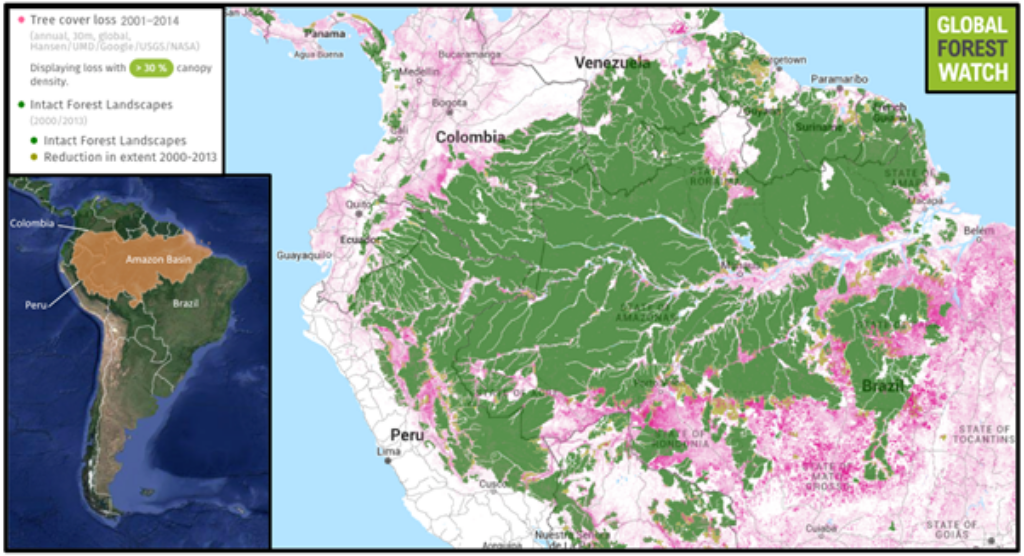
In Tocantins, Brazil’s newest state established in 1988, agricultural deforestation is rapidly escalating between 1977 and 2008, Tocantins experienced a 66% reduction in its forest cover.For example, deforestation can lead to reduced rainfall, affecting both water supply and agricultural productivity, which in turn impacts food security and health.(CDKN, 2016)
In 2012, hydroelectric power represented 76.9% of Brazil’s electricity matrix, making it the country’s primary energy source. While hydroelectricity has well-documented benefits, this situation highlights the hydrological regime’s sensitivity to environmental changes, particularly the decreasing discharge in the basin due to alterations in precipitation. On average, projected scenarios indicate an extended duration of low discharge levels within the annual hydrological cycle. The sensitivity of discharge, as reflected by precipitation elasticity, is expected to increase as a result of climate change impacts. (Mohor, 2015)
2.2. Socio-economic conditions
In the late 19th and early 20th centuries, migration played a key role in establishing new urban centers, initially along waterways and later along federal roads built in the 1960s. In Brazil, urban areas grew significantly along highways connecting Belém, Brasília, Cuiaba, Porto Velho, and Rio Branco. However, many areas in the Amazon Basin remain poorly connected, with transportation and communication primarily along the Amazon River. (UNEP 2004)
By 1996, the population in the Amazon Basin had grown to 6.7 million, reflecting high growth rates in Brazil’s North and Middle-West regions. Urbanization in the Basin increased from 60.8% in 1991 to 70.7% in 1996. Despite this growth, the North region lags in infrastructure and social investment, leading to a lower quality of life compared to other South American regions. (UNEP 2004)
The 20th century saw increased government intervention in the Amazon, with policies aimed at promoting economic development and national integration. The construction of roads and infrastructure opened up the region to further exploitation, leading to deforestation, agricultural expansion, and the emergence of new settlements. This period also saw the rise of large-scale industrial activities, such as mining and hydropower development, further impacting the environment and local communities (UNEP 2004)
The population is concentrated in urban centers, with inadequate water and sewage treatment creating chronic public health issues. Water pollution increases infant mortality due to severe diarrhea and promotes the spread of endemic and tropical diseases. Poor drinking water quality and insufficient sewage treatment are the main health problems associated with pollution, particularly in urban areas. (UNEP 2004)
2.3. Technological innovation
For the last two decades,the Amazon Basin has long been a battleground between conservationists seeking to protect its vast forests and those advocating for resource-intensive development. While deforestation rates have declined in recent years, a new paradigm is emerging: the Amazonia Third Way (A3W). This initiative aims to reconcile environmental protection with economic development by harnessing the potential of the Fourth Industrial Revolution (4IR). (Nobre, 2018)
The 4IR, characterized by advancements in artificial intelligence, biotechnology, and digital technologies, offers unprecedented opportunities to unlock the value of the Amazon’s biodiversity. By leveraging these technologies, A3W envisions creating a “green economy” that supports bio-industries and local development while safeguarding the rainforest and its indigenous inhabitants. This approach echoes historical patterns of technological innovation driving economic growth, such as the Industrial Revolution’s impact on manufacturing and the digital revolution’s transformation of information and communication. A3W aims to harness the transformative power of the 4IR to create a sustainable future for the Amazon, balancing conservation with economic prosperity. (Nobre, 2016)
Technological advancements have played a significant role in shaping the Amazon’s socio-ecological dynamics. The introduction of new agricultural technologies, such as mechanized farming and the use of fertilizers, has increased agricultural productivity but has also contributed to soil degradation and water pollution. Similarly, the development of hydroelectric dams, while providing energy, has disrupted natural river flows, impacting biodiversity and local communities. The introduction of modern infrastructure, such as roads and highways, has facilitated access to the region’s resources but has also contributed to deforestation and habitat fragmentation.
2.4. Governance structure
The Amazon Basin has experienced a shifting governance landscape, characterized by periods of both centralized and decentralized control. During the colonial era, the Portuguese crown implemented a centralized governance structure, prioritizing resource extraction and economic development over environmental conservation. This approach led to significant deforestation and land degradation, setting the stage for future challenges. In the post-colonial period, Brazil adopted a more decentralized approach, devolving authority to regional and local governments. However, this shift has not always been effective in addressing the complex environmental challenges faced by the region. (UNEP 2004)
In the early 2000s, increasing deforestation in the Brazilian Amazon highlighted the ineffectiveness of previous control initiatives. To address this, the Ministry of the Environment (MMA) proposed a reorganization of Federal Government activities, aiming to establish a new economic development model that incorporated sustainability.
The MMA’s approach operated on three fronts:
- A sustainable development program for the macro-region, committing Federal and State funds to Brazil’s Sustainable Amazon Program (PAS).
- An action plan (PPCDAm) for immediate interventions to reverse deforestation rates.
- A local development plan for regions most threatened by deforestation, employing multi-actor, multi-sector, and multi-level governance strategies.
These initiatives, launched between 2003 and 2004, involved various ministries and related agencies, emphasizing the need for intersectoral actions and political support from the Presidency. Strategies were based on five key premises:
- Elevating sustainability as a government-wide matter.
- Ensuring political solidity and internal summoning power.
- Promoting intersectoral commitments across ministries and agencies.
- Establishing a permanent evaluation system for policies.
- Building an external support community for continuity.
This integrated governance approach aimed to address social inequality, environmental degradation, and promote sustainable economic growth in the Amazon region. (Larrea, 2025)
2.5. Climatic factors
The Amazon Basin is highly sensitive to climate change, experiencing increased temperatures, altered rainfall patterns, and more frequent extreme weather events. The Amazon Basin’s rainfall patterns are significantly influenced by the El Niño/Southern Oscillation (ENSO) and the North Atlantic Oscillation (NAO). These effects are further influenced by a 28-year precipitation cycle, with the most significant floods occurring when La Niña coincides with the wet phase of this cycle, and the worst droughts, like the 1992 drought, when El Niño coincides with the dry phase. The NAO also affects the region, contributing to severe droughts such as the 2005 event, which led to record-low river levels in southern and western tributaries. These climatic factors create complex and variable precipitation patterns in the Amazon Basin.
These changes have significant implications for the region’s water resources, agricultural productivity, and human health. Rising temperatures and altered rainfall patterns have increased the risk of droughts and floods, impacting water availability and agricultural yields. Climate change has also contributed to the spread of diseases, such as malaria and dengue fever, which pose a significant threat to human health.
Critical Transition and Inflection Point: A Framework for Analysis
3.1. Mid-20th Century Urbanization Surge
This period saw a profound shift from a predominantly rural, resource-based economy to a rapidly urbanizing landscape, marked by significant population growth, infrastructure development, and economic diversification.
The Rubber Era (early 20th century): Cities emerged as vital hubs for the rubber trade, acting as collection points for latex flowing downstream to global markets and supply centers for labor and resources needed for extraction activities deep within the basin.(Richard, 2015)
Shift from Extractive to Industrial Economies: The mid-20th century witnessed a move away from traditional extractive industries like rubber and timber towards more industrial activities like mining, hydroelectric power generation, and large-scale agriculture. This transition spurred significant economic growth but also triggered ecological changes and social disruptions.(Richard, 2015)
Population Shift from Rural to Urban Areas: The expansion of industrial activities and infrastructure led to a mass migration from rural areas to newly established urban centers. This demographic shift fundamentally altered the social fabric of the region, creating new urban landscapes and exacerbating existing social inequalities.(Richard, 2015)
Increased Integration into Global Markets: The Amazon Basin became increasingly integrated into global markets during this period, driven by rising demand for its resources and the emergence of new trade routes. This integration brought about economic benefits but also introduced vulnerabilities to external economic fluctuations and environmental pressures (Richard, 2015)
The path dependency is the mid-20th century urbanization surge, driven by industrialization and resource extraction. This rapid urbanization, fueled by the demand for rubber and timber, led to the development of infrastructure, transportation networks, and settlements. However, it also contributed to deforestation, pollution, and the displacement of indigenous populations. This initial trajectory set the stage for subsequent transitions, shaping the region’s resource management practices, land use patterns, and population distribution.
3.2. 1970s Agricultural Transformation 1970s
The 1970s marked a critical transition in the Amazon basin’s agricultural systems and food security trajectories, setting the stage for the complex dynamics observed today. This period witnessed a confluence of factors that significantly altered the region’s agricultural landscape and its relationship with food security.
Crop Yield Trends and Agricultural Intensification: The Green Revolution, with its focus on high-yielding varieties and chemical inputs, reached the Amazon in the 1970s. This led to a surge in crop yields, particularly for staples like rice and soybeans. However, this intensification came at a cost. The reliance on fertilizers and pesticides, coupled with monoculture practices, contributed to soil degradation, water pollution, and biodiversity loss. The expansion of agricultural frontiers, driven by the demand for these crops, also led to widespread deforestation, further impacting the region’s delicate ecosystems.( Ickowitz, 2018).
Dietary Shifts and Nutrition Transitions: As incomes rise and urbanization progresses, there is an increasing demand for processed foods, animal products, and imported goods. This dietary shift has led to increased consumption of calorie-dense foods and a decline in the consumption of traditional, nutrient-rich foods. This dietary transition has implications for nutrition and health, potentially leading to an increase in diet-related chronic diseases ( Ickowitz, 2018).
The 1970s Agricultural Transformation, marked by the expansion of large-scale cattle ranching and soy production, further solidified this path dependency. Government policies incentivized deforestation for agricultural purposes, leading to widespread land clearing and a shift towards monoculture practices. This transformation not only intensified pressure on the Amazon’s ecosystems but also fostered a dependence on resource-intensive agricultural models, with significant implications for water availability, soil health, and biodiversity.
3.3. Public Health Dynamics and Environmental Change
A critical inflection point in the Amazon basin’s health history occurred in the mid-20th century, coinciding with the acceleration of deforestation and industrial development. This period saw a dramatic increase in vector-borne diseases like malaria and dengue, as well as emerging zoonotic infections (Tucker, 2017).
This shift marked a transition from a relatively stable, albeit challenging, disease landscape to one characterized by rapid and unpredictable changes. This historical grounding is crucial for scenario modeling because it demonstrates how environmental changes can drive disease emergence and alter the effectiveness of traditional public health interventions. Understanding the specific factors that contributed to this inflection point, such as the expansion of agriculture, and road construction, provides valuable insights for designing future interventions that address the interconnectedness of health, environment, and development in the Amazon (Ellwanger, 2020)
The Amazon Basin’s public health trajectory, intricately intertwined with environmental change, exemplifies the concept of path dependency. The mid-20th century marked a critical inflection point, where the acceleration of deforestation and industrial development triggered a dramatic shift in the disease landscape. This shift, characterized by a surge in vector-borne diseases and emerging zoonotic infections, wasn’t a random occurrence; it was a consequence of the path set in motion by earlier decisions and trends (Tucker, 2017).
This historical trajectory, characterized by the interconnectedness of development, environmental change, and disease emergence, has created a complex path dependency. The legacy of deforestation, coupled with the expansion of agricultural activities and infrastructure development, has significantly altered the Amazon’s ecosystem, rendering it more susceptible to disease outbreaks. This path dependency is further reinforced by the emergence of new zoonotic infections, a direct consequence of human encroachment into previously undisturbed ecosystems.
The Amazon’s public health challenges are not isolated incidents but rather the outcome of a historical process. The region’s current health status is a reflection of past decisions and actions. Understanding this path dependency is crucial for informing future interventions, as it highlights the need for integrated approaches that consider the interconnectedness of health, environment, and development. Addressing public health issues in the Amazon requires not only tackling immediate health challenges but also addressing the underlying drivers of environmental change, such as unsustainable agricultural practices and infrastructure development. Failure to recognize and address this path dependency will likely perpetuate the cycle of disease emergence and hinder efforts to achieve sustainable development in the region.
3.4. Water Availability and Management Histories
Decades of hydrological data reveal a concerning trend of increased variability in rainfall patterns and river flows within the Amazon basin. Exceptional hydrological events in the last decades, such as the floods in 2009, 2012, and 2014, and the droughts in 2005 and 2010, have significantly impacted the region, alerting scientists, governments, and the general public to the impacts of climate variability. There is a significant impact of rainfall changes on inundation levels in the Amazon basin. Using a basin-wide hydrological model, Melack and Coe found that a 10% to 25% decrease in rainfall led to a corresponding reduction in inundation, ranging from -5% to -20% and -12% to -30%, respectively (Sorribas, 2016).
The Amazon Basin is subject to the regulations of two significant international agreements. The Amazon Cooperation Treaty, signed in Brasilia, Brazil on July 3, 1978, and effective as of August 2, 1980, lays the groundwork for cooperation among the countries sharing the Amazon River. This treaty was later strengthened by the Amendment Protocol, adopted on December 14, 1998, which led to the formation of the Amazon Cooperation Treaty Organization. This organization serves as a dedicated platform for promoting collaborative efforts in managing the Amazon Basin’s vast resources (IW: LEARN).
The basin’s water resources are vital for hydropower generation, supporting diverse ecosystems, and sustaining agricultural production. However, the increasing demand for these resources has led to conflicts over water allocation, putting pressure on ecosystems and human health. Climate change further complicates the nexus, impacting rainfall patterns, river flows, and the frequency and intensity of droughts and floods (International Water Governance)
The Amazon Basin’s water management is stuck in a cycle of unsustainable practices, a legacy of historical decisions prioritizing resource extraction and economic growth over environmental protection. This path dependency, driven by powerful economic interests and limited capacity for change, makes it increasingly difficult to implement sustainable water management practices, even as the Amazon faces the growing threats of climate change and deforestation.
3.5. Energy Evolution and Infrastructure Legacies
The Amazon Basin’s energy landscape is dominated by fossil fuels, primarily oil and gas extraction, which contribute significantly to the region’s economy. However, this dependence poses significant environmental and social risks.
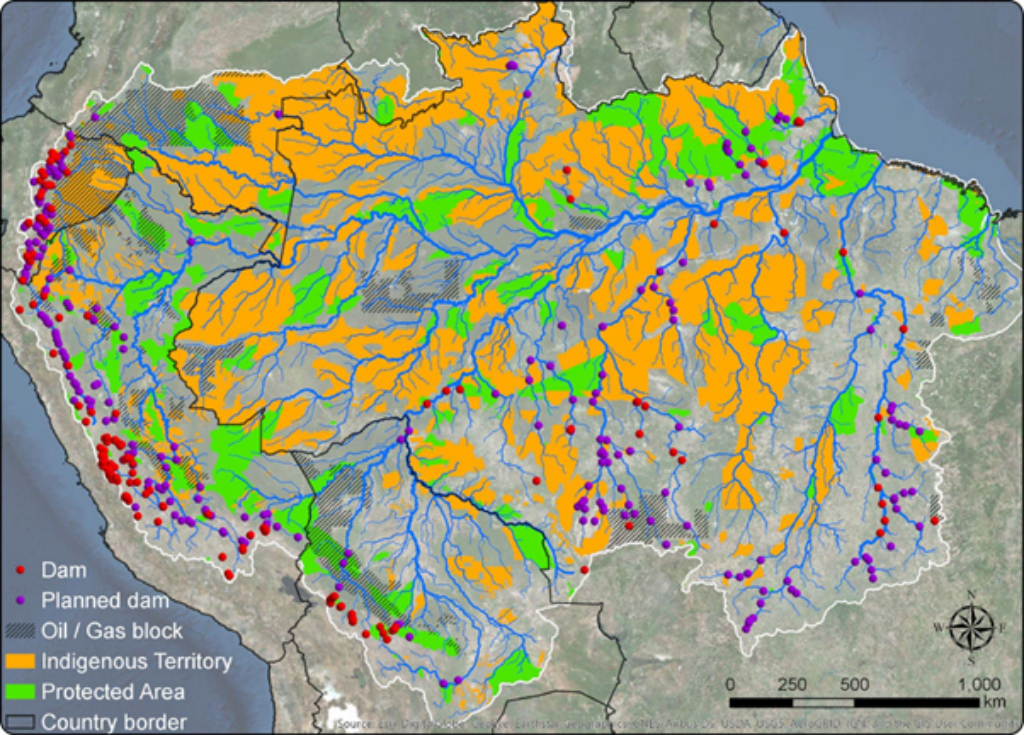
Figure 4: The location of fossil fuels reserves and dams in the Amazon Basin. Data sources: protected areas (Brazilian Ministry of the Environment, Ecuadorian Ministry of the Environment, Colombian Ministry of Environment and Sustainable Development, SIGOT Colombia, Peruvian Servicio Nacional de Áreas Naturales Protegidas por el Estado [SERNANP], GeoBolivia, and Servicio Nacional de Áreas Protegidas); Indigenous territories (Instituto SocioAmbiental, Rede Amazônica de Informação Socioambiental Georreferenciada [RAISG], GeoBolivia, and SIGOT Colombia); oil and gas blocks (Brazil Agência Nacional do Petróleo, Bolivia Agencia Nacional de Hidrocarburos, SIGOT Colombia, and Finer et al. 2015); dams (Brazil Agência Nacional de Energia Elétrica).
Between 2010 and 2012, electricity generation and transmission (mainly hydropower) led to the reduction or downgrading of 19 conservation units or protected areas. The extraction process often leads to deforestation, habitat fragmentation, and pollution, jeopardizing the fragile ecosystem. Furthermore, the burning of fossil fuels contributes to climate change, exacerbating the already vulnerable state of the Amazon rainforest. (Finer, 2015)
The region’s infrastructure development, including roads, pipelines, and hydroelectric dams, has been driven by the need to facilitate resource extraction and economic growth. While infrastructure expansion offers economic benefits, it also introduces vulnerabilities. Water is abundant in the Amazon, but access to clean drinking water and sanitation is limited. Roads, for instance, can lead to increased deforestation and habitat loss, while dams can disrupt river ecosystems and alter water flow patterns. These infrastructure projects often involve large-scale land acquisition and displacement of indigenous communities, raising social and ethical concerns (Schaeffer,2023)
The Amazon Basin’s energy landscape is locked in a cycle of unsustainable development, a legacy of historical decisions prioritizing resource extraction and economic growth over environmental and social well-being. This path dependency, deeply rooted in the region’s infrastructure and economic interests, perpetuates a vicious cycle of environmental degradation and social injustices.
The region’s initial focus on fossil fuels, primarily oil and gas extraction, established a powerful economic paradigm that continues to shape development choices. This reliance on fossil fuels, while contributing to economic growth, has come at a significant environmental cost, fueling deforestation, habitat fragmentation, and pollution. The burning of fossil fuels further exacerbates climate change, threatening the already vulnerable Amazon rainforest.
The pursuit of economic development has also driven the construction of extensive infrastructure, including roads, pipelines, and hydroelectric dams. While these infrastructure projects offer economic benefits, they also introduce vulnerabilities and disrupt delicate ecosystems. Roads, for instance, facilitate deforestation and habitat loss, while dams disrupt river ecosystems and alter water flow patterns. The construction of these projects often involves large-scale land acquisition and displacement of indigenous communities, raising significant social and ethical concerns.
This path dependency has created a strong inertia, making it difficult to shift towards more sustainable energy sources and development models. Powerful economic interests, entrenched in the fossil fuel industry and infrastructure development, resist changes that could threaten their profits and influence. Furthermore, limited capacity and financial resources in many Amazonian countries hinder the implementation of alternative solutions.
Breaking free from this path dependency requires a fundamental shift in priorities, prioritizing environmental sustainability and social justice over short-term economic gains. This shift necessitates a comprehensive approach that includes:
- Transitioning to renewable energy sources: Investing in renewable energy technologies like solar, wind, and hydro power, while ensuring responsible development practices that minimize environmental impacts.
- Strengthening environmental regulations: Implementing robust regulations to protect the Amazon rainforest, reduce deforestation, and mitigate pollution from fossil fuel extraction.
- Promoting sustainable land management practices: Supporting sustainable agriculture, forestry, and ecotourism that preserve biodiversity and ecosystem services.
- Protecting indigenous rights: Ensuring the participation and consent of indigenous communities in development projects, respecting their land rights and cultural heritage.
The Amazon Basin’s future hinges on breaking free from this unsustainable path dependency. By embracing a sustainable development model that prioritizes environmental protection, social equity, and long-term prosperity, the region can unlock its full potential and ensure a brighter future for its people and ecosystems.
3.6. Climate Variability and Anthropogenic Influences
The Amazon Basin is experiencing a complex interplay of climate variability and anthropogenic influences, pushing the system towards potential tipping points
Climate Variability: The Amazon Basin is highly sensitive to climate variability, particularly rainfall patterns. There’s been an increase in mean air temperature of 0.6°C- 0.7°C over the last 40 years, with 2016 being the warmest year (0.9°C ± 0.3°C), followed by 1998. Droughts, like the 2005 and 2010 events, triggered significant ecological changes, impacting forest health, biodiversity, and ecosystem services. These events serve as potential inflection points, highlighting the vulnerability of the system to climate extremes (Marengo, 2018)
Anthropogenic Influences: Deforestation, land-use change, and unsustainable agricultural practices are exacerbating the effects of climate variability. Deforestation reduces evapotranspiration, altering regional rainfall patterns and increasing drought risk (CDKN, 2016). Land-use change alters water flow and sediment transport, impacting river ecosystems and biodiversity. These anthropogenic pressures can push the system towards critical thresholds, accelerating the pace of change ( Davidson, 2012).
The Amazon Basin is a complex system with a history of human interaction. Deforestation, land-use change, and unsustainable agricultural practices have been ongoing for decades, leading to a gradual degradation of the forest ecosystem. These activities have altered the landscape, impacting water flow, nutrient cycling, and biodiversity. As a result, the Amazon Basin is now more vulnerable to climate variability, as evidenced by the increased frequency and intensity of droughts.
The increasing vulnerability of the Amazon Basin to climate variability has led to a cycle of degradation. Droughts are more likely to occur due to deforestation and climate change, and these droughts further exacerbate the effects of deforestation by reducing forest resilience and increasing the risk of forest fires. This cycle is self-reinforcing, leading to a path-dependent trajectory towards a tipping point.
The Amazon Basin is at a critical juncture. The path dependency created by deforestation and climate change has increased the vulnerability of the system, making it more susceptible to tipping points. Urgent action is needed to mitigate climate change, reduce deforestation, and promote sustainable land-use practices to prevent the Amazon Basin from crossing a tipping point and experiencing irreversible degradation.
Conclusion
The Amazon Basin, a global treasure trove of biodiversity and ecosystem services, faces a complex interplay of forces that are shaping its future. The historical analysis reveals a tapestry of interwoven trends and transitions, highlighting the profound impact of past choices on the region’s current state. From the mid-20th century urbanization surge to the 1970s agricultural transformation, each critical transition has left its mark on the region’s resources, infrastructure, and human well-being.
Understanding these path dependencies is not merely an academic exercise; it is essential for informed decision-making. By acknowledging the interconnectedness of water, food, energy, health, and climate, we can navigate towards a future where the Amazon Basin thrives. This requires a shift towards sustainable practices, adaptive governance, and a commitment to preserving the ecological integrity of this vital region.
The insights gained from this study provide a robust foundation for scenario modeling and policy interventions. By understanding the past, we can empower stakeholders to make informed decisions that promote resilience, ensure the well-being of future generations, and safeguard the Amazon’s irreplaceable contribution to the global ecosystem.
The Case of Tana River Basin, Kenya
Daisy Awuor
Introduction
Tana River Basin contributes to 32% of Kenya’s overall river runoff and its management falls under the Water Resources Authority (WRA), formerly known as the Water Resources Management Authority (WRMA) (Suda,2024). The Tana River is the main river in the basin and it originates in the flanks of Mount Kenya in Tharaka County and discharges its contents into the Indian Ocean at the Tana Delta (Gitau, 2023). The largest and most important uses of this water system remain to be commercial irrigation and hydroelectric power generation, with the networks of dams such as Masinga, Kiambere, Gitaru, Kamburu, and Kindaruma utilising the flow in the Tana River and its tributaries to generate electricity at a capacity of 567 MW (Koei, 2013). The Mangrove forests in the Tana River Basin are also noted for their unique ecosystem services, essential in supporting the livelihoods of people living and dependent on the coastal resources (Gitau, 2023).
Historical Water Governance
Management planning for the Tana River Basin is important to maximize the benefits derived from ecosystem goods and services and avoid resource use conflicts. Several policies have been implemented, including;
Tana Delta Integrated Management Plan (2017-2027)
The overall goal of the Tana Delta Intergrated Management Plan is to manage the Tana delta ecosystem and resources sustainably towards ecological integrity and socioeconomic development for the present and future generations.Implementation of the management plan to achieve its overall goal and management objectives is guided by the Public participation and inclusive approach, Polluter pays principle and Ecosystem approach in management (Nema, 2017). Public participation and inclusive approach is an integrated approach that entails the involvement of relevant stakeholders and consensus building on matters of planning and decision-making.The Polluter pays principle implies that persons who pollute the delta’s wetlands should meet the cost of cleaning them up, restoring, rehabilitating and also meet the cost of the pollution to resource users while the ecosystem approach in management activities recognizes the relationship and inter-linkage between ecological and socio-economic components of the Delta 60.
Tana River Delta Land Use Plan (2015)
Developed through a collaborative effort led by Nature Kenya this plan involves regulated access, wise use and improved rangeland management that will lead to improved sustainable livelihoods, security and equity, and biodiversity conservation in the Tana River Basin. The Land Use Plan aims to harmonize competing land uses by providing a framework that supports government, private sector, and smallholder developments. Effective implementation of the Land Use Plan involves zoning the delta for multiple land uses, restricting degrading developments to already impacted areas, and conducting environmental impact assessments for proposed alterations (Odhengo, 2014). This approach aims to maintain the ecological integrity of the delta while supporting socio-economic development.
Climate Variability and Anthropogenic Influences
Climate Variability
The Tana River Basin has experienced significant climate variability, with dry events dominating from 1960 to 1980 and wet events prevalent from 1990 to 2000 (Polong, 2019) leading to reduced agricultural productivity, food insecurity, and environmental degradation. Fluctuations in streamflow and the decreased frequency but increased intensity of rainfall events are driven by climatic phenomena such as the Indian Ocean Dipole (IOD) and El Niño Southern Oscillation (ENSO) (Kiptum, 2024) which are also associated with short rains from October to December that affect water availability during these months. These variations lead to recurrent flooding and prolonged droughts, exacerbating food insecurity and poverty among local communities (Karienye & Macharia, 2021).
The yearly rainfall patterns show a bimodal trend with two main rainfall seasons: the first and the long rainfall season starts in April and peaks in May (MAM), while the second and short rainfall season is from October to December (OND), with its peak in November. The long rains (MAM) have nearly twice the amplitude of the short rains (OND) and contribute to 46.5 % of the total annual rainfall. Over the next four decades, there is a potential for a substantial rise in mean sea level by 15–25 cm, which could severely limit agricultural activities in the lower floodplain areas within 20 to 30 km of the coast (Odhengo, 2014). Tidal surges transport saltwater up to 30 km inland from the River Tana’s mouth, posing threats to livelihoods such as fishing and farming.
Anthropogenic Influences
The largest and most important uses of the Tana River Basin water system remain to be commercial irrigation and hydroelectric power generation, with increased demand following the 35% increase in population between 1999 and 2009. Collective use of water for hydroelectric power production, irrigation, domestic consumption, commercial and industrial establishments and pastoralism exert a huge demand on the water system.(Odhiambo-Ochieng, 2016) The modification of the River Tana flow regime has manifested in freshwater shortage downstream causing long periods of drought, considerable constraints on irrigation activities and confrontations between pastoralists and crop farmers over grazing areas and watering points leading to loss of lives.
Agricultural Systems and Food Security
Agriculture is the main socio-economic activity influencing water abstraction in the Tana River Basin. The Masinga Horticulture Irrigation Project (12,000 ha) and Kiambere Fruit Irrigation Project (150 ha) are part of the government irrigation development schemes. The Upper Tana River catchment supports smallholder farming systems, where soil fertility is enhanced through organic resources like cattle manure and leguminous plants. Private irrigation schemes, coffee, horticulture and floriculture are intensively practised in Muranga, Thika, Lower Kiambu and Nyeri using sprinkler irrigation (Nganga, 2020). Along the main Tana River, rice, bananas and fruit irrigation schemes exist. In the lower Tana basin, livestock farming is the main socio-economic activity. Small-scale crop production along the river floodplain is practised by the Pokomo who grow maize, bananas and mangoes. Other socio-economic activities that support livelihood include fishing and small-scale businesses.
Unsustainable agricultural practices characterized by slash and burn agriculture and overgrazing are leading to degradation of land resources. Large tracts of land within the delta have been set aside for large scale agriculture projects and for mining exploration by the government. This includes the Tana Delta Irrigation Project (TDIP) land owned by TARDA among others. (Nunow, 2011) In addition, settlements have taken up some important dry season grazing areas within the delta and communities from outside the pastoral areas have settled in these areas and started crop farming. Promoting sustainable practices such as efficient irrigation techniques, crop diversification, and agroforestry can reduce water usage and enhance food security. These practices also contribute to climate resilience and support biodiversity conservation
Hydroelectric power generation
The Tana River Basin has five major reservoirs built between 1968 and 1987, crucial for hydropower, irrigation, and drinking water supply. The basin hosts the Seven Forks project, a system of five hydropower plants supplying around 70% of Kenya’s electricity. (Hurford, 2014) Key reservoirs include Masinga, Kiamburu, and Kiambere (Hunink, 2013). The Masinga Reservoir regulates streamflow, ensuring efficient water allocation for power generation while protecting downstream ecology during varying inflow conditions (Oludhe, 2013).Recent severe droughts in 2005, 2006, and 2009 highlighted vulnerabilities, necessitating water and electricity rationing. Operating the hydropower dams to support economic development without reducing hydropower generation, water supplies or ecological function is a challenge. Recent advancements focus on optimizing reservoir management strategies to balance energy generation, irrigation demands, and ecosystem services.
Public Health Dynamics and Environmental Change
Analysis of water from the Tana River, during the rainy season, has yielded coliform counts of above 100/100ml and E. coli values greater than 10/100ml, which is indicative of pollution by sewage. Water pollution results from contaminated stormwater discharges, and poor disposal of urban and industrial wastes that leach or seep and only eventually end up into the water system.Increased farming coupled with the proliferation of industries and urban centres along the Tana Basin has contributed to the pollution of the water resource. Effluent from upstream-based tea factories and chemical wastes from mechanized agricultural farms (Mwea, Bura, Hola and Garsen irrigation schemes) are also affecting water quality (Nema, 2017). Towns and settlements along the river course in the counties of Muranga, Kiambu, Nyeri, Kirinyaga, Embu, Garrisa and Tana River Counties are polluting the river because they lack functional sewerage systems.Poor water quality can also result in health issues, including waterborne diseases, which disproportionately affect vulnerable populations. Moreover, the competition for water among different users can exacerbate tensions and inequalities, further complicating efforts to achieve food security. (UNEP,2016)
Conclusion
The Tana River Basin faces severe land-use and water systems pressures due to increase in agricultural land, urbanization and poor watershed management that threaten biodiversity and water security. Implementing integrated policy frameworks that consider the interdependencies of the water-energy-food (WEF) nexus in the Tana River Basin can lead to more effective resource management and sustainable outcomes. To ensure long-term sustainability, policies must strengthen watershed management frameworks by integrating climate-resilient agricultural techniques and land-use planning. Governance mechanisms should empower local communities in decision-making processes, ensuring that land use aligns with ecological capacity. Policies should aim at addressing disparities, supporting marginalized groups, and upholding the right to development. Additionally, investments in climate adaptation strategies, such as reforestation and soil conservation, must be prioritized to mitigate drought impacts and enhance water retention.
The Case of Rhine River Basin
(Switzerland, Austria, Liechtenstein, Germany, France, The Netherlands)
Grace Jimenez
Background
The Rhine river is an important river in Central Europe pivoting a total of 1233 km of longitude. Its catchment directly interacts with nine countries: Austria, Belgium, France, Germany, Italy, Liechtenstein, Luxemburg, The Netherlands and Switzerland. While it is not part of the Eurasian rivers with the most discharge, ranking 9th, it is an artery for the most important economic regions of Europe. In regards to its direct link with civil society, the human population of the basin equals 58 millions individuals, from which many live in the areas surrounding the river margin. The Rhine has been labeled as a multipurpose waterway, catering transportation, power generation, industrial production, urban sanitation, drinking water for 25 million people, agriculture and tourism. Due to this role, it has greatly influenced the events of the European continent through its development. However, because of industrialization and rapid urbanization, its ecological and biodiversity dimensions have been greatly compromised (Uehlinger et al., 2009).
Geographical and Socioeconomic Overview
The geography of the basin has been changing depending on external factors, remarkably due to the presence of humanity. As this river has been entangled in the history of Europe, playing an important role in the Roman occupation, the founding of Rotterdam, the Saint-Elisabeth flood, and others, its evolution can potentially be seen as a reflection of the state of society (Ultrecht University, 2025). Due to the exchange of resources that took place across the Rhine River during the second half of the 19th century, the basin grew rapidly with the construction of ports and roads.
Two industries were highly developed in this period: heavy industry and chemical industry. For the chemical industry, the Rhine served two functions: the transportation of raw materials, and cooling and a waste water deposit. It is for this reason that, globally, the Rhine basin is one of those with the highest density of industrial plants, therefore with a high concentration of pollutants. It should be noted that considerable pollution has decreased since the late 1970s due to the implementation of the ICPR Rhine Action Programme, thus, water quality has increased considerably (International Commission for the Protection of the Rhine, 2025). As for fishing activity, during the 19th century the basin represented the biggest producer of salmon in the world, promoting intensive fishery. Later, in the 20th century the construction of several hydropower dams, notably the Basel dam, altered the migratory routes of fauna and significantly polluted the surrounding stream. Today, fishing represents a minor activity due to the shortage of fishes. For example, in the upper Rhine, there are only two commercial fishing companies left, in addition to 80 artisanal fishermen in the same area (Cramer et al., 2014).
Progressive changes in the basin
Progressively, due to the region’s urbanization and industrialization processes, many modern cities consolidated along the basin, as the closeness to a water body guaranteed an easier access to fuel and trade. The rising need for water in different sectors not only influenced the region’s development, but also shaped the physical shape of the basin, evidencing a link between the geography aspects of the river and the socio-economic dynamics entangled with it (Hooijer et al., 2004). In the present day, availability of water has taken more importance as in the past. In order to maintain the availability of this resource, projects such as dam construction, water diversion, and land-use changes were initiated. This took more importance as the climatic change modified the rainy seasons, therefore, the crop production had to be rearranged in accordance to the availability. As projections until 20100 suggest, wetter winters and drier summers are to be expected. This is not only due to the faster melting of the snow, but also because of the growing glacier retreat. This is the reason why having resource management for the long-term becomes crucial (Swiss Academy of Sciences, 2025).
Water Availability and Management Histories
The Rhine basin has been historically fed by precipitation and meltwater from glaciers. The steady level of discharges had made it a steady source of water in the past, however, its availability has been threatened by the changes due to climate change. For instance, the glaciers retreat, making the amount of meltwater less each year; precipitation patterns change, with less precipitation during the summer; snow has become a less frequent element for the river’s water source; and rain has become more usual as the temperatures have risen. These factors have altered the availability of water, making it scarce in seasons in which it is more needed, like summer (Deltares, 2025). Moreover, not only the quantity of water is at stake, but also the quality of the resource. As there is leftover water available, the pollutants tend to concentrate more, as well as giving a shorter time frame for their dilution.
Agriculture and food security
Historically, the basin’s water resources are specially in use during dry seasons, in which surface irrigation is prioritized (Schoot, 2021). While the water quantity destined to irrigate crops has been steady in the long run, the basin is still subject to changes in its capacity due to external components and natural changes. Eventually, climate change started to concern food production. Because of the changes in precipitation amounts, the risk of flooding increases. Consequently, the choice for land use, choices of crops and cropping calendar need to be modified in accordance to these new available resources. Another negative effect from this change in timeframes is the lower yield potentials due to a shortened growing season. This is further compromised by the increased amount of CO2 in crop water, which also lowers the quality and growth potential of crops, highlighting the importance of the correct management of the Rhine for correct food production and security (Silvis & Van Der Heide, 2013).
Energy Evolution and Infrastructure
The potential of the Rhine basin for energy had a peak during the Industrial Revolution period, when it was both used for transportation of resources and cooling of energy-producing systems. In regards to transportation, the river is a major corridor for petroleum transportation and it is also a connector between the major refineries, from which the ARA is highlighted (Amsterdam, Rotterdam and Antwerp). Due to the lack of the region’s petroleum product pipeline infrastructure and high-price of other transportation options, such as railways, a dependence on the Rhine becomes tangible for this sector. Furthermore, several surrounding countries benefit from the basin. For instance, due to its origins in the Swiss Alps, Switzerland benefits greatly from the generation of energy; second of all, France and Germany also hold hydropower plants in the basin due to belonging to the Upper Rhine, best scenario for hydroelectric centrals; finally, The Netherlands benefits from less hydropower potential, nonetheless, it still takes advantage of tidal and wind energy (Mangold & Hamman, 2013).
Climate Change Trends and Impacts
Historically, the Rhine River’s climate varies by season, having the warmest months during summer, mild periods during spring and autumn, and cold winters. While the temperature in summer peaks at 25°C, the temperature during winter is close to freezing. In regards to the months with the most extreme temperatures, January is the coldest month, with a change of snow, while Juy is the hottest one (CRUI, 2025). This pattern has been always steady, however, due to the rise of water temperature led by warmer air temperatures, changes in the temperatures per season are expected to happen in the following years (International Commission for the Protection of the Rhine, 2025). In addition to the expected change of climate patterns due to global warming, extreme weather events have a higher probability to happen in this context. Normally, these events would not be non-existent, as there is always a risk. However, during the most recent years these probabilities have risen in consequence of a higher water level, meaning floods, and higher temperatures during dry seasons, meaning droughts.
In addition to these trends, invasive species are constantly expanding through navigation channels. Historically, these have generally been invertebrates, however since 2006 the migration has also included fish. These changes have modified the dynamics between prey and predators, altering the biological quality of resources (International Commission for the Protection of the Rhine, 2015). It is highly probable that the river will not be able to return to its original state despite improvement attempts, nonetheless, it is important to try to mitigate the adverse ecological effects to stabilize the existing species diversity.
To face these emerging issues, adaptation strategies and policy responses have been taken by concerned stakeholders. The International Commission for the Protection of the Rhine (ICPR) is undertaking transboundary measures, such as levee improvements, floodplain restoration, and retention basins, to mitigate extreme weather events. Such measures complement the early warning systems and climate monitoring to improve preparedness. Furthermore, policy responses, led by the same organization, include much integration with the EU Water Framework Directive and Floods Directive, thus standing for cross-border cooperation. This will subsequently be enhanced through sustainable land use, sediment management, and stakeholder engagement.
Conclusion
In conclusion, the Rhine River basin has evolved over the centuries and has been mostly shaped by human activities (economic activities, urbanization, social dynamics, etc). Furthermore, the river basin’s exposure to pollution from different economic sectors has been steadily growing, which hindered its capacity to successfully supply the civil society. Moreover, the river has been greatly concerned about the impact of climate change. For instance, only by the rising water level, the risk of floods becomes greatly compromised. In essence, a non-proper management of the basin could potentially temporarily stop certain economic activities, such as commerce and fishery.
Bibliography:
- Asian Development Bank (ADB) 1996, Greater Mekong Subregion Economic Cooperation Program: Promoting Development through Connectivity, ADB, Manila.
- Bestari, N, Levy, P & Anul, S 2013, ‘Hydropower development in the Mekong region: Risks, opportunities, and sustainability challenges’, Energy Policy, vol. 52, pp. 643-657.
- CDKN Global (2016) The water-energy-food nexus: Insights from amazonia | climate & development knowledge network. Available at: https://cdkn.org/story/water-energy-food-amazonia (Accessed: 27 February 2025).
- Clean Water Program (2016) Squarspace. Available at: https://static1.squarespace.com/static/5845a70859cc6819f2dfdb9e/t/5a1da039f9619afa6aba2a54/1511891007355/Clean+Water+Prog+1-pager+2016.pdf (Accessed: 08 March 2025).
- Cramer, W.A., Yamashita, E., Baniulis, D., Whitelegge, J. and Hasan, S.S., 2014. Structure–function of the cytochrome b6f complex of oxygenic photosynthesis. In: W.J. Lennarz and M.D. Lane, eds. Encyclopedia of Biological Chemistry. 2nd ed. Elsevier, pp. 329–334. Available at: https://doi.org/10.1016/B978-0-12-369449-2.00006-0[Accessed 9 March 2025].
- CRUI, 2025. Weather on the Rhine River. CRUI. Available at: https://www.crui.se/blog/weather-on-the-rhine-river/#:~:text=The%20Rhine%20River’s%20climate%20varies,near%20freezing%2C%20especially%20in%20January [Accessed 9 March 2025].
- Davidson, E.A. (2012) (PDF) the amazon basin in transition. Available at: https://www.researchgate.net/publication/221760309_The_Amazon_Basin_in_transition (Accessed: 06 March 2025).
- Delgado, L, Thaler, T & Seher, W 2020, ‘Integrated Water-Energy-Food Nexus management in the Mekong Basin: A framework for sustainable development’, in Proceedings of the International Water Resources Conference, UNESCO, Paris, pp. 214-228.
- Deltares, 2025. Climate change and the consequences for water supplies in Europe. Deltares. Available at:https://www.deltares.nl/en/news/climate-change-and-the-consequences-for-water-supplies-in-europe [Accessed 9 March 2025].
- DuBowy, P.J. (2013) ‘Mississippi River Ecohydrology: Past, present and future’, Ecohydrology & Hydrobiology, 13(1), pp. 73–83. doi:10.1016/j.ecohyd.2013.02.003.
- Ellwanger, J.H. et al. (2020) Beyond diversity loss and climate change: Impacts of amazon deforestation on infectious diseases and public health, Anais da Academia Brasileira de Ciências. Available at: https://www.scielo.br/j/aabc/a/fRVhxyPq4NLCsKTZPJMzV8J/ (Accessed: 07 March 2025).
- Environmental Encyclopedia (2025) .” environmental encyclopedia. . encyclopedia.com. 10 Feb. 2025 ., Encyclopedia.com. Available at: https://www.encyclopedia.com/environment/encyclopedias-almanacs-transcripts-and-maps/amazon-basin (Accessed: 28 February 2025).
- Federal Water Pollution Control Admin Twin Cities-Upper Mississippi River Project (1964) Summary and Pollution Abatement Recommendations For The Upper Mississippi River and Major Tributaries. NEPIS.
- Finer, M. et al. (2015) IOPscience, Environmental Research Letters. Available at: https://iopscience.iop.org/article/10.1088/1748-9326/10/2/024003?utm_source=wiley&getft_integrator=wiley (Accessed: 07 March 2025).
- Gitau, P., Duvail, S. and Verschuren, D., 2023. Evaluating the combined impacts of hydrological change, coastal dynamics and human activity on mangrove cover and health in the Tana River delta, Kenya. Regional Studies in Marine Science. Available at: https://doi.org/10.1016/j.rsma.2023.102898 [Accessed 9 March 2025].
- Guo, Q. (2023) ‘Strategies for a resilient, sustainable, and equitable Mississippi River Basin’, River, 2(3), pp. 336–349. doi:10.1002/rvr2.60.
- History of the Hypoxia Task Force (2025) EPA. Available at: https://www.epa.gov/ms-htf/history-hypoxia-task-force (Accessed: 08 March 2025).
- Hooijer, A., Klijn, F., Pedroli, G. and Os, [no first name], 2004. Towards sustainable flood risk management in the Rhine and Meuse river basins: Synopsis of the findings of IRMA-SPONGE. River Research and Applications, 20(3), pp. 20. Available at: https://doi.org/10.1002/rra.781 [Accessed 9 March 2025].
- Hunink, J.E., Niadas, I.A., Antonaropoulos, P., Droogers, P. and de Vente, J., 2013. Targeting of intervention areas to reduce reservoir sedimentation in the Tana catchment (Kenya) using SWAT. Hydrological Sciences Journal – Journal Des Sciences Hydrologiques, 58(3), pp. 600–614. Available at: https://doi.org/10.1080/02626667.2013.774090[Accessed 9 March 2025].
- Hurford, A., Hurford, A., Harou, J.J. and Harou, J.J., 2014. Balancing ecosystem services with energy and food security – Assessing trade-offs from reservoir operation and irrigation investments in Kenya’s Tana Basin. Hydrology and Earth System Sciences, 18(8), pp. 3259–3277. Available at: https://doi.org/10.5194/HESS-18-3259-2014 [Accessed 9 March 2025].
- Ickowitz (2018) Agricultural intensification, dietary diversity, and markets in the Global Food Security Narrative, Global Food Security. Available at: https://www.sciencedirect.com/science/article/pii/S2211912418300099 (Accessed: 06 March 2025).
- International Commission for the Protection of the Rhine (ICPR), 2025. Agriculture. ICPR. Available at:https://www.iksr.org/en/topics/uses/agriculture [Accessed 9 March 2025].
International Commission for the Protection of the Rhine (ICPR), 2025. Climate change in the Rhine catchment. ICPR. Available at: https://www.iksr.org/en/topics/climate-change-in-the-rhine-catchment [Accessed 9 March 2025]. - International Commission for the Protection of the Rhine, 2015. The biology of the Rhine: Summary report on the Rhine measurement programme biology 2012/2013 and national assessments according to the WFD (Report No. 232). Available at: https://www.iksr.org/fileadmin/user_upload/DKDM/Dokumente/Fachberichte/EN/rp_En_0232.pdf[Accessed 9 March 2025].
- International Commission for the Protection of the Rhine, 2025. Industry. IKSR. Available at:https://www.iksr.org/en/topics/uses/industry [Accessed 9 March 2025].
- International Waters Governance (no date) Amazon Basin, International Waters Governance. Available at: http://www.internationalwatersgovernance.com/amazon-basin.html (Accessed: 07 March 2025).
- IW:LEARN (no date) Learn: Documents -> legal frameworks -> amazon basin, IW. Available at: https://iwlearn.net/documents/legal-frameworks/amazon-basin (Accessed: 07 March 2025).
- Karienye, D. and Macharia, J.M., 2021. Adaptive Capacity to Mitigate Climate Variability and Food Insecurity of Rural Communities Along River Tana Basin, Kenya. In: Springer, Cham, pp. 49–60. Available at: https://doi.org/978-3-030-45106-6_57 [Accessed 9 March 2025].
- Kibii, J.K., Kipkorir, E.C. and Kosgei, J.R., 2021. Application of Soil and Water Assessment Tool (SWAT) to Evaluate the Impact of Land Use and Climate Variability on the Kaptagat Catchment River Discharge. Sustainability, 13(4), p. 1802. Available at: https://doi.org/10.3390/SU13041802 [Accessed 9 March 2025].
- Kiptum, A., Guigma, K., Antonarakis, A.S. and Todd, M.C., 2023. Characteristics, Drivers, and Predictability of Flood Events in the Tana River Basin, Kenya. Available at: https://doi.org/10.2139/ssrn.4582970 [Accessed 9 March 2025].
- Kuenzer, C, Campbell, I, Roch, M, Leinenkugel, P, Tuan, VQ & Dech, S 2012, ‘Understanding the impact of hydropower developments in the context of climate change in the Lower Mekong Basin’, Environmental Science & Policy, vol. 25, pp. 89-99.
- Larrea C (2025) Amazon Assessment Report 2021, The Amazon We Want – The Amazon We Want. Available at: https://www.theamazonwewant.org/amazon-assessment-report-2021/ (Accessed: 04 March 2025).
- Lauri, H, Moel, H, Ludwig, F, Supit, I, Kummu, M & Ward, P 2012, ‘Future changes in Mekong River hydrology: Impact of climate change and reservoir operation on discharge’, Journal of Hydrology, vol. 464–465, pp. 233–245.
- Mangold, M. and Hamman, P., 2013. Renewable energies in the Upper Rhine Region from a stakeholder viewpoint: Projects, territorialisation and networks in the making. Wageningen University & Research. Available at:https://doi.org/10.4324/9781003199977 [Accessed 9 March 2025].
- Marengo, J.A. et al. (2018) Changes in climate and land use over the Amazon region: Current and future variability and Trends, Frontiers. Available at: https://www.frontiersin.org/journals/earth-science/articles/10.3389/feart.2018.00228/full (Accessed: 07 March 2025).
- Mekong River Commission (MRC) 2018, State of the Basin Report 2018, MRC Secretariat, Vientiane.
- Mississippi River between Missouri River & Minneapolis (2024) Rock Island District. Available at: https://www.mvr.usace.army.mil/about/offices/programs-and-project-management/district-projects/projects/article/1168713/mississippi-river-between-missouri-river-minneapolis/ (Accessed: 08 March 2025).
- Mississippi River Gulf of Mexico Watershed Nutrient Task Force (2008) Gulf Hypoxia Action Plan 2008: For reducing mitigating, and controlling hypoxia in the northern Gulf of Mexico and improving water quality in the Mississippi River Basin. Washington, D.C: U.S. Environmental Protection Agency, Office of Wetlands, Oceans, and Watersheds.
- Mohor, G.S. et al. (2015) Exploratory analyses for the assessment of climate change impacts on the energy production in an Amazon run-of-river hydropower plant, Journal of Hydrology: Regional Studies. Available at: https://www.sciencedirect.com/science/article/pii/S2214581815000324 (Accessed: 28 February 2025).
- Molle, F, Foran, T & Kakonen, M 2009, ‘Contested waterscapes in the Mekong region: Hydropower, livelihoods and governance’, Water Alternatives, vol. 2, no. 1, pp. 55-78.
- Murtoff, J. (2025) Amazon Basin, Encyclopædia Britannica. Available at: https://www.britannica.com/place/Amazon-Basin (Accessed: 28 February 2025).
- Mwaguni, S., Ruwa, R., Ochiewo, J. and Osore, M., 2016. Integrated Water Resources Management in a Changing Climate: The Implications of Anthropogenic Activities on the Tana and Athi/Sabaki Rivers Water System. Available at: http://dx.doi.org/10.1007/978-3-319-25370-1_7 [Accessed 9 March 2025].
- National Research Council, Division on Earth and Life Studies, Water Science and Technology Board and Committee on the Mississippi River and the Clean Water Act (2008) ‘Characteristics of the Mississippi River System’, in Mississippi River Water Quality and the Clean Water Act: Progress, Challenges, and Opportunities. National Academies Press, pp. 23–24. Available at: https://books.google.co.ma/books?id=gBBkAgAAQBAJ&lpg=PA21&dq=mississippi%20river&lr&hl=fr&pg=PA24#v=onepage&q=mississippi%20river&f=false.
- Nema, 2017. Tana Delta Integrated Management Plan. NEMA. Available at:https://www.nema.go.ke/images/Docs/Management_Plans/Tana%20delta%20Management%20plan%202017-27-min.pdf [Accessed 9 March 2025].
- Nganga, W.B., Ng’etich, K.O., Macharia, M.J., Kiboi, N.M., Adamtey, N. and Ngetich, K.F., 2020. Multi-influencing-factors’ evaluation for organic-based soil fertility technologies out-scaling in Upper Tana Catchment in Kenya. 7. Available at: https://doi.org/10.1016/J.SCIAF.2019.E00231 [Accessed 9 March 2025].
- Nitrogen and Phosphorus Pollution in the Mississippi River Basin (2011) EPA. Available at: https://nepis.epa.gov/Exe/ZyPURL.cgi?Dockey=P100MBKF.txt (Accessed: 08 March 2025).
- Nobre, C.A. et al. (2016) Land-use and climate change risks in the Amazon and the need of a novel Sustainable Development Paradigm, Proceedings of the National Academy of Sciences of the United States of America. Available at: https://pmc.ncbi.nlm.nih.gov/articles/PMC5047175/ (Accessed: 28 February 2025).
- Nobre, I. and Nobre, C.A. (2018) The Amazonia Third Way Initiative: The Role of Technology to unveil the potential of a novel tropical biodiversity-based economy, IntechOpen. Available at: https://www.intechopen.com/chapters/63918 (Accessed: 04 March 2025).
- Plastic Pollution in the Mississippi River – Regional Cooperation for a Transboundary Problem (2021) Trash Free Waters Article Series [Preprint]. doi:https://www.epa.gov/sites/default/files/2021-05/documents/trash_free_waters_mississippi_river_plastics_article.pdf.
- Pukinskis, I, Bravard, J & Ziv, G 2019, ‘Sediment dynamics and impacts of dam construction on the Mekong River system’, Geomorphology, vol. 327, pp. 23-39.
- Richards, P. and VanWey, L. (2015) Where deforestation leads to urbanization: How resource extraction is leading to urban growth in the Brazilian Amazon, Annals of the Association of American Geographers. Association of American Geographers. Available at: https://pmc.ncbi.nlm.nih.gov/articles/PMC4789292/ (Accessed: 06 March 2025).
- Schaeffer, R. (2023) A new infrastructure for the Amazon. Available at: https://www.theamazonwewant.org/wp-content/uploads/2023/12/PB-Infraestructure-EN-nov23.pdf (Accessed: 07 March 2025).
- Schoot, F.H.E. van, 2021. Estimating current and possible future irrigation water requirements: An approach for the Rhine basin during the growing season in periods of drought. Master’s thesis, Wageningen University & Research.
- Silvis, H.J. and van der Heide, C.M., 2013. Policy framework for climate-smart agriculture: Assessment of policies and market developments in the Netherlands. Wageningen University & Research. Available at:https://edepot.wur.nl/361901 [Accessed 9 March 2025].
- Sorribas, M.V. (2016) (PDF) projections of climate change effects on discharge and inundation in the Amazon Basin. Available at: https://researchgate.net/publication/297675385_Projections_of_climate_change_effects_on_discharge_and_inundation_in_the_Amazon_basin (Accessed: 07 March 2025).
- Surveillance and Analysis Division (1972) Industrial pollution of the lower mississippi river in Louisiana. Dallas, TX: United States Environmental Protection Agency, Surveillance and Analysis Division.
- Swiss Academy of Sciences, 2025. ASG II: Synthesis report on climate change impacts in Switzerland. Swiss Academy of Sciences. Available at: https://portal-cdn.scnat.ch/asset/9dbdfe41-a991-526b-a0af-86fab9925907/ASG-II_Synthese_EN_mit-Links.pdf?b=49f8141a-6ebb-5651-895e-fff50cf0c7db&v=e513446a-c901-5aa5-aa7b-d4b89bb654bc_0&s=PyVA2ZNkoExH9SNknnHKd6vDDcSM86j5XFn0dphYiV91z2yTnlvH_A9a4_WvDvEL9QH1PixAmXJYaiGJgF29-1VwbSeCIlQ92NssoPkpYJLvXTQmyQkcLjRduKnhfEYgVXegX0QBiJYRIq3R_UVGJ70YDmClacHxJL4uiSU_ZAE[Accessed 9 March 2025].
- The Mississippi/Atchafalaya River Basin (MARB) (no date a) EPA. Available at: https://www.epa.gov/ms-htf/mississippiatchafalaya-river-basin-marb (Accessed: 08 March 2025).
- The National Academy of Sciences (2007) Mississippi River water quality and the Clean Water Act: Available at: https://nap.nationalacademies.org/resource/12051/miss_river_cwa_final.pdf (Accessed: 08 March 2025).
- Tucker, J.M. (2017) Does deforestation promote or inhibit malaria transmission in the Amazon? A systematic literature review and critical appraisal of current evidence | philosophical transactions of the royal society B: Biological Sciences. Available at: https://royalsocietypublishing.org/doi/10.1098/rstb.2016.0125 (Accessed: 07 March 2025).
- Turner, R.E. and Rabalais, N.N. (1991) ‘Changes in Mississippi River Water Quality this century’, BioScience, 41(3), pp. 140–147. doi:10.2307/1311453.
- Uehlinger, U., Wantzen, K., Leuven, R.S.E.W. and Arndt, H., 2009. The Rhine River Basin. In: K. Tockner, U. Uehlinger and C.T. Robinson, eds. Rivers of Europe. Academic Press, pp. 199–245. Available at:https://doi.org/10.1016/B978-0-12-369449-2.00006-0 [Accessed 9 March 2025].
- UNEP (2004) Amazon Basin. Available at: https://wedocs.unep.org/bitstream/handle/20.500.11822/8402/-Amazon%20Basin%20-%20GIWA%20Regional%20Assessment%2040B-20043741.pdf?sequence=3&isAllowed=y (Accessed: 28 February 2025).
- United Nations Economic and Social Commission for Asia and the Pacific (UNESCAP) 2019, The Mekong River Basin: A Regional Review, United Nations, Bangkok.
- Utrecht University, 2025. Maps reveal the historical path of the Rhine. Utrecht University. Available at:https://www.uu.nl/en/news/maps-reveal-the-historical-path-of-the-rhine [Accessed 9 March 2025].
- World Bank 2010, The Nam Theun 2 Hydropower Project: A Case Study of Environmental and Social Management, World Bank Publications, Washington, DC.
- World Wide Fund for Nature (WWF) 2020, The Mekong River Basin in Crisis: Hydropower, Deforestation, and Climate Change, WWF, Gland.
- Xu, X & Grumbine, RE 2014, ‘Transboundary river governance and the challenges of hydropower development in the Mekong’, in Proceedings of the International River Basin Management Conference, Bangkok, pp. 53-68.
Discover more from Nexus Journals
Subscribe to get the latest posts sent to your email.